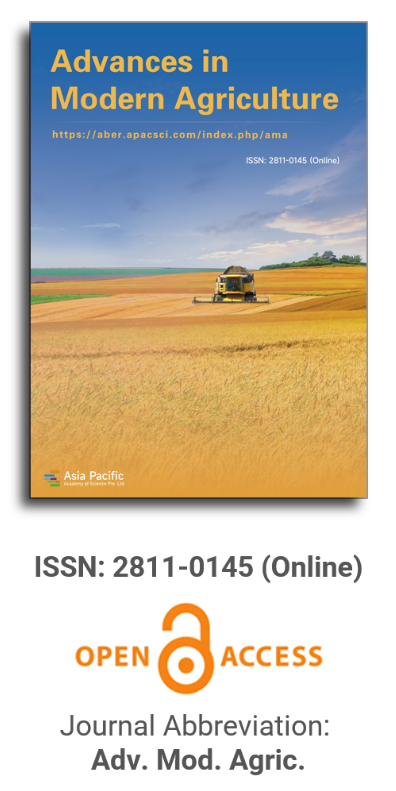
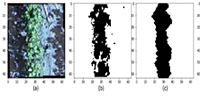
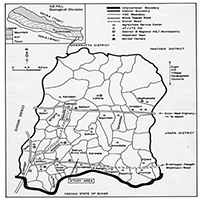
Unveiling hybrid potential and exploring combining ability for yield and related traits in maize (Zea mays L.) through line × tester mating design
Vol 5, Issue 4, 2024
VIEWS - 2143 (Abstract)
Download PDF
Abstract
Combining ability analysis serves as an invaluable tool for evaluating the compatibility of parental lines and testers, as well as for elucidating the intricate genetic mechanisms at play within their hybrid progeny. This study was designed to ascertain the combining ability of maize lines when paired with testers, specifically focusing on yield-related traits through the utilization of a line × tester mating design. A total of fifteen advanced lines were systematically crossbred with three distinct testers to produce forty-five hybrid test crosses. The performance of these progenies was rigorously assessed across three distinct locations, thereby enhancing the robustness of the findings. The field trials were conducted using an alpha lattice design. Variance analysis, combining ability effects, and genetic components were estimated following a line × tester analysis. Employing variance analysis, significant variations were discerned in both general and specific combining abilities, underscoring the contribution of both additive and non-additive gene actions to the expression of the targeted traits. Notably, the magnitudes variance component indicated the prevalence of additive gene effects across the traits studied. Amidst the comprehensive exploration of parental lines and testers, it was evident that lines L10 and Tester T2 exhibited notable compatibility as general combiners, particularly in the context of maize grain yield. Additionally, Line L12 demonstrated favorable characteristics related to earliness. The superior performance of certain hybrid combinations emerged as a noteworthy outcome of this investigation. Specifically, the hybrid cross L10 × T2 displayed remarkable performance in terms of grain yield, while L12 × T1 demonstrated strong potential for the trait days to anthesis. Furthermore, in terms of specific combining ability, the cross L13 × T1 demonstrated the most pronounced effect, particularly concerning grain yield. Following closely were the combinations L5 × T1 and L2 × T2, each exhibiting significant potential for enhancing maize productivity. To conclude, this study underscores the indispensable role of combining ability analysis in elucidating the interplay between parental lines and testers, thus unraveling the intricate genetic dynamics within their hybrid offspring. The insights gathered hold promise for advancing maize production by employing judicious selection strategies, with a specific focus on the highlighted hybrid combinations.
Keywords
References
- Piperno DR, Flannery KV. The earliest archaeological maize (Zea mays L.) from highland Mexico: New accelerator mass spectrometry dates and their implications. In: Proceedings of the National Academy of Sciences; 2001.
- Khakwani K, Cengiz R, Asif M, Ahsan M. Heterotic and heritability pattern of grain yield and related traits in doubled haploid f1 hybrids of maize (Zea mays l.). Maydica; 2020.
- Mogesse W, Zelleke H, Nigussie M. General and Specific Combing Ability of Maize (< i> Zea mays L.) Inbred Line for Grain Yield and Yield Related Traits Using 8×8 Diallel Crosses. American Journal of BioScience. 2020; 8(3): 45. doi: 10.11648/j.ajbio.20200803.11
- Prasanna BM. Global maize R4D scenario: Challenges and opportunities in Asia. In: Proceedings of the 12th Asian Maize Conference and Expert Consultation on Maize for Food, Feed, Nutrition and Environmental Security; Bangkok, Thailand; 2014.
- FAOSTAT. Statistical Database. Food and Agriculture Organization of the United Nations, Rome, Italy. Available online: http://fenix.fao.org/faostat/internal/en/#data (accessed on 13 November 2024).
- Fischer T, Byerlee D, Edmeades G. Crop yields and global food security. Australian Centre for International Agricultural Research; 2014.
- Zivanovic T, Vuckovic S, Prodanovic S, et al. Evaluation of inbred lines as sources of new alleles for improving elite maize hybrid. Cereal Research Communications. 2006; 34(2-3): 941-948. doi: 10.1556/crc.34.2006.2-3.223
- Rezaei A, Yazdisamadi B, Zali A, et al. Estimate of heterosis and combining ability in maize (Zea mays L.) using diallel crossing method. Agricultural and Food Sciences; 2004.
- Liaqat S, Hidayat R, Asif A, et al. Combining ability estimates from line x tester mating design in maize (Zea mays l). Academic J Agricultural Science and Research. 2015;3: 71-75.
- Smith CAB, Kempthorne O. An Introduction to Genetic Statistics. Journal of the Royal Statistical Society Series A (General). 1958; 121(3): 358. doi: 10.2307/2342912
- Matzinger DF. Comparison of Three Types of Testers for the Evaluation of Inbred Lines of Corn1. Agronomy Journal. 1953; 45(10): 493-495. doi: 10.2134/agronj1953.00021962004500100010x
- Qu Z, Li L, Luo J, et al. QTL Mapping of Combining Ability and Heterosis of Agronomic Traits in Rice Backcross Recombinant Inbred Lines and Hybrid Crosses. PLoS ONE. 2012; 7(1): e28463. doi: 10.1371/journal.pone.0028463
- Bhatnagar S, Betrán FJ, Rooney LW. Combining Abilities of Quality Protein Maize Inbreds. Crop Science. 2004; 44(6): 1997-2005. doi: 10.2135/cropsci2004.1997
- Betrán FJ, Isakeit T, Odvody G. Aflatoxin Accumulation of White and Yellow Maize Inbreds in Diallel Crosses. Crop Science. 2002; 42(6): 1894-1901. doi: 10.2135/cropsci2002.1894
- Glover MA, Willmot DB, Darrah LL, et al. Diallel Analyses of Agronomic Traits Using Chinese and U.S. Maize Germplasm. Crop Science. 2005; 45(3): 1096-1102. doi: 10.2135/cropsci2004.0493
- Kang MS, Zhang Y, Magari R. Combining Ability for Maize Weevil Preference of Maize Grain. Crop Science. 1995; 35(6): 1556-1559. doi: 10.2135/cropsci1995.0011183x003500060006x
- Kim SK, Ajala SO. Combining ability of tropical maize germplasm in West Africa. II. Tropical vs temperate x temporal origins. Maydica; 1996.
- Alam M, Rahman M, Ahmed S, et al. Genetic Variation and Genotype by Environment Interaction for Agronomic Traits in Maize (Zea mays L.) Hybrids. Plants. 2022; 11(11): 1522. doi: 10.3390/plants11111522
- Snedecor GW, & Cochran WG. Statistical methods, 8th ed. Iowa: Iowa State University Digital Press; Ames, IA, USA; 1989.
- Kempthorne O. An introduction to genetic statistics. New York: John Wiley & Sons, Inc.; London: Chapman & Hall Ltd.; 1957.
- Rodríguez F, Alvarado G, Pacheco Á, et al. AGD-R (Analysis of genetic designs with R for Windows) version 4.0. International Maize and Wheat Improvement Center (CIMMYT). CIMMYT Research Data & Software Repository Network; 2015.
- Alemnesh A. Test cross performance and combining ability studies of elite maize (Zea mays L.) inbred lines in the central rift valley of Ethiopia. Jimma University; 2012.
- Amiruzzaman M, Islam MA, Hassan L, Rohman MM. Combining Ability and Heterosis for Yield and Component Characters in Maize. Academic Journal of Plant Sciences. 2010.
- Gissa DW, Zelleke H, Labuschagne MT, et al. Heterosis and combining ability for grain yield and its components in selected maize inbred lines. South African Journal of Plant and Soil. 2007; 24(3): 133-137. doi: 10.1080/02571862.2007.10634795
- Gudeta T, Gurmu N. Heterosis and combining abilities in QPM versions of early generation highland maize (Zea mays l.) Inbred lines. Ethiopia; 2007.
- Hadji TH. Combining ability analysis for yield and yield-related traits in quality protein maize (QPM) inbred lines. M. Sc. School of graduate studies; 2004.
- Jemal A. Heterosis and combining ability for yield and related traits in maize (Zea mays L.). Alemaya University; 1999.
- Teshale A. Analysis of tropical Highland Maize (Zea mays L.) Inbred lines top crossed with three east African Populations. Alemaya University; 2001.
- Aly R, Amer E. Combining Ability and Type of Gene Action for Grain Yield and Some Other Traits Using Line X Tester Analysis in Newly Yellow Maize Inbred Lines (Zea mays L.). Journal of Plant Production. 2008; 33(7): 4993-5003. doi: 10.21608/jpp.2008.166840
- Camdzija Z, Filipovic M, Vancetovic J, et al. The evaluation of combining abilities for grain yield of Zp maize inbred lines using the line × tester analysis. In: Proceedings of the third International Scientific Symposium Agrosym Jahorina 2012; 2012.
- Sofi PA, Rather AG, Dar Z. Association of Heterotic Expression for Grain Yield and its Component Traits in Maize (Zea mays L.). International Journal of Agricultural Research. 2007; 2(5): 500-503. doi: 10.3923/ijar.2007.500.503
- Ahmad A, Saleem M. Combining ability analysis in maize. International Journal of Agricultural and Biological. 2003.
- Legesse BW, Pixley KV, Botha AM. Combining ability and heterotic grouping of highland transition maize inbred lines. Maydica; 2009.
- Mosa HE. Estimation of Combining Ability of Maize Inbred Lines Using Top Cross Mating Design. J Agric Res Kafer El-Sheikh Univ. 2010;36: 1-16.
- Pswarayi A, Vivek BS. Combining ability amongst CIMMYT’s early maturing maize (Zea mays L.) germplasm under stress and non-stress conditions and identification of testers. Euphytica. 2007; 162(3): 353-362. doi: 10.1007/s10681-007-9525-0
- Zeleke H, Tuna H. Combining ability analysis for yield and related traits in quality protein maize (Zea mays L.) inbred lines. International Journal of Biological Sciences. 2010;2: 2010-2015.
- Aminu D, Izge AU. Heritability and Correlation Estimates in Maize (Zea mays L.) Under Drought Conditions in Northern Guinea and Sudan Savannas of Nigeria. World Journal of Agricultural Sciences. 2012.
- Abrha SW, Zeleke HZ, Gissa DW. Line x tester analysis of maize inbred lines for grain yield and yield related traits. Asian Journal of Plant Science and Research. 2013.
- Elmyhum M. Estimation of combining ability and heterosis of quality protein maize inbred lines. African Journal of Agricultural Research. 2001;8: 6309-6317.
- Fan XM, Chen HM, Tan J, et al. Combining abilities for yield and yield components in maize. Maydica; 2008.
- Kambe GR, Udaykumar K, Lohithaswa HC, et al. Combining ability studies in maize (Zea mays L). Mol Plant Breed. 2013;4: 116-127.
- Betrán FJ, Ribaut JM, Beck D, et al. Genetic Diversity, Specific Combining Ability, and Heterosis in Tropical Maize under Stress and Nonstress Environments. Crop Science. 2003; 43(3): 797-806. doi: 10.2135/cropsci2003.7970
- Gebre GB. Genetic variability and inheritance of drought and plant density adaptive traits in maize. Agricultural and Food Sciences, Biology; 2005.
- Pal SS, Khera AS, Dhillon BS. Genetic analysis and selection advance in maize population. Maydica; 1986.
- Sandesh GM, Karthikeyan A, Kavithamani D, et al. Heterosis and combining ability studies for yield and its component traits in Maize (Zea mays L.). Electronic Journal of Plant Breeding. 2018; 9(3): 1012. doi: 10.5958/0975-928x.2018.00126.6
- Shengu MK, Gissa DW, Zelleke H, Tilahun L. Combining ability analysis of early maturing maize (Zea mays L.) inbred lines in central rift valley of Ethiopia. International Journal of Scientific and Research Publications. 2016.
- Amiruzzaman M, Amin MN. Evaluation of inbred lines of field corn through line × tester method. Gazipur, Bangladesh; 2011.
- De Rissi R, Hallauer AR. Evaluation of four testers for evaluating maize (Zea mays L.) lines in a hybrid development program. Rev Bras Genet. 1991;14: 467-481.
- Talukder MZA, Banik B. Evaluation of inbred lines through line × tester method. Gazipur, Bangladesh; 2012.
- Reif JC, Gumpert FM, Fischer S, et al. Impact of Interpopulation Divergence on Additive and Dominance Variance in Hybrid Populations. Genetics. 2007; 176(3): 1931-1934. doi: 10.1534/genetics.107.074146
- Springer NM, Stupar RM. Allele-Specific Expression Patterns Reveal Biases and Embryo-Specific Parent-of-Origin Effects in Hybrid Maize. The Plant Cell. 2007; 19(8): 2391-2402. doi: 10.1105/tpc.107.052258
- Zhan W, Cui L, Yang S, et al. Natural variations of heterosis-related allele-specific expression genes in promoter regions lead to allele-specific expression in maize. BMC Genomics. 2024;25: 1-11. doi:10.1186/S12864-024-10395-Y/FIGURES/6 https://doi.org/10.1186/s12864-024-10395-y
- Springer NM, Stupar RM. Allelic variation and heterosis in maize: How do two halves make more than a whole? Genome Research. 2007; 17(3): 264-275. doi: 10.1101/gr.5347007
- Dong Y, Li G, Zhang X, et al. Genome-Wide Association Study for Maize Hybrid Performance in a Typical Breeder Population. International Journal of Molecular Sciences. 2024;25: 1190. doi:10.3390/IJMS25021190/S1
Refbacks
- There are currently no refbacks.
Copyright (c) 2024 Mohammad Ashraful Alam, Salahuddin Ahmed, Mohammad Alamgir Miah, Nasrin Jahan, Mohammad Marufur Rahman, Mohammad Rafiqul Islam
License URL: https://creativecommons.org/licenses/by/4.0/
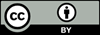
This site is licensed under a Creative Commons Attribution 4.0 International License (CC BY 4.0).
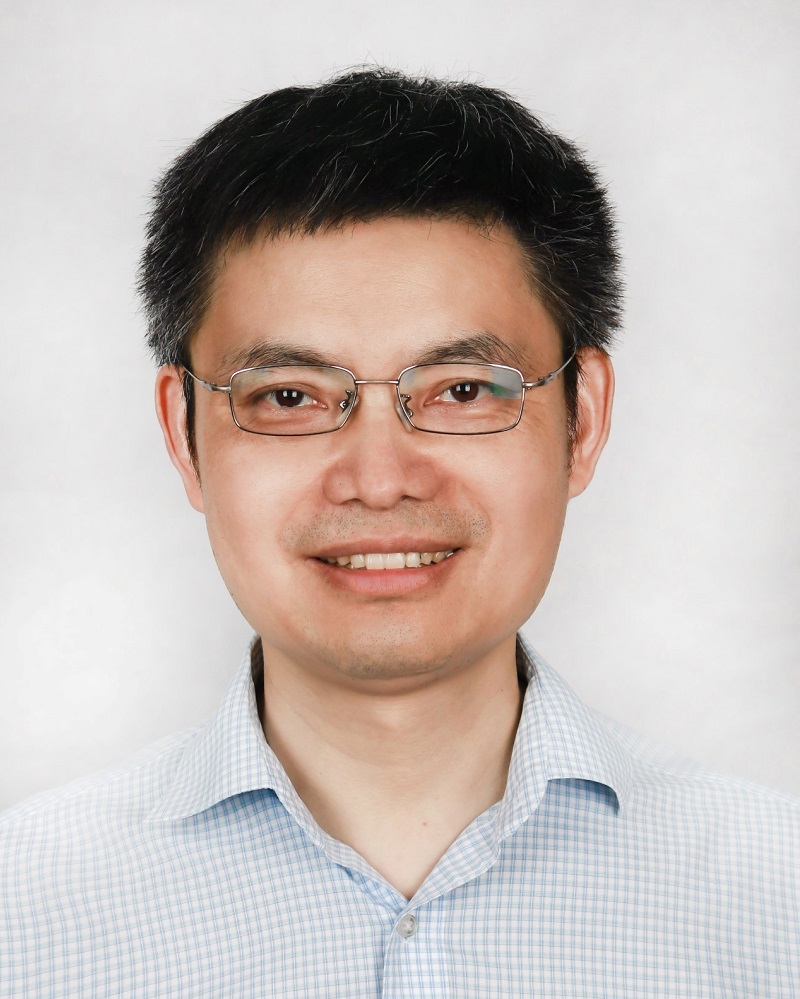
Prof. Zhengjun Qiu
Zhejiang University, China
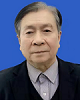
Cheng Sun
Academician of World Academy of Productivity Science; Executive Chairman, World Confederation of Productivity Science China Chapter, China
Indexing & Archiving
In the realm of modern agriculture, the integration of cutting-edge technologies is revolutionizing the way we approach sustainable farming practices. A recent study published in Advances in Modern Agriculture titled "Classification of cotton water stress using convolutional neural networks and UAV-based RGB imagery" has garnered significant attention for its innovative approach to precision irrigation management. Conducted by researchers from Institute of Data Science and the AgriLife Research and Extension Center of Texas A&M University (authors's information is below). This study introduces a novel method for classifying cotton water stress using unmanned aerial vehicles (UAVs) and convolutional neural networks (CNNs), offering a powerful solution for optimizing water use in agriculture.
Modern agricultural technology is evolving rapidly, with scientists collaborating with leading agricultural enterprises to develop intelligent management practices. These practices utilize advanced systems that provide tailored fertilization and treatment options for large-scale land management.
This journal values human initiative and intelligence, and the employment of AI technologies to write papers that replace the human mind is expressly prohibited. When there is a suspicious submission that uses AI tools to quickly piece together and generate research results, the editorial board of the journal will reject the article, and all journals under the publisher's umbrella will prohibit all authors from submitting their articles.
Readers and authors are asked to exercise caution and strictly adhere to the journal's policy regarding the usage of Artificial Intelligence Generated Content (AIGC) tools.
Asia Pacific Academy of Science Pte. Ltd. (APACSCI) specializes in international journal publishing. APACSCI adopts the open access publishing model and provides an important communication bridge for academic groups whose interest fields include engineering, technology, medicine, computer, mathematics, agriculture and forestry, and environment.