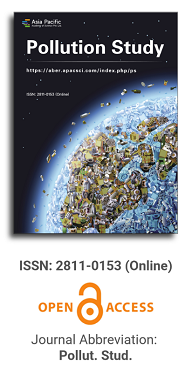
Asia Pacific Academy of Science Pte. Ltd. (APACSCI) specializes in international journal publishing. APACSCI adopts the open access publishing model and provides an important communication bridge for academic groups whose interest fields include engineering, technology, medicine, computer, mathematics, agriculture and forestry, and environment.
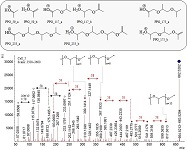
The (partial) replacement of synthetic polymers with bioplastics is due to increased production of conventional packaging plastics causing for severe environmental pollution with plastics waste. The bioplastics, however, represent complex mixtures of known and unknown (bio)polymers, fillers, plasticizers, stabilizers, flame retardant, pigments, antioxidants, hydrophobic polymers such as poly(lactic acid), polyethylene, polyesters, glycol, or poly(butylene succinate), and little is known of their chemical safety for both the environment and the human health. Polymerization reactions of bioplastics can produce no intentionally added chemicals to the bulk material, which could be toxic, as well. When polymers are used to food packing, then the latter chemicals could also migrate from the polymer to food. This fact compromises the safety for consumers, as well. The scarce data on chemical safety of bioplastics makes a gap in knowledge of their toxicity to humans and environment. Thus, development of exact analytical protocols for determining chemicals of bioplastics in environmental and food samples as well as packing polymers can only provide warrant for reliable conclusive evidence of their safety for both the human health and the environment. The task is compulsory according to legislation Directives valid to environmental protection, food control, and assessment of the risk to human health. The quantitative and structural determination of analytes is primary research task of analysis of polymers. The methods of mass spectrometry are fruitfully used for these purposes. Methodological development of exact analytical mass spectrometric tools for reliable structural analysis of bioplastics only guarantees their safety, efficacy, and quality to both humans and environment. This study, first, highlights innovative stochastic dynamics equations processing exactly mass spectrometric measurands and, thus, producing exact analyte quantification and 3D molecular and electronic structural analyses. There are determined synthetic polymers such as poly(ethylenglycol), poly(propylene glycol), and polyisoprene as well as biopolymers in bags for foodstuffs made from renewable cellulose and starch, and containing, in total within the 20,416–17,495 chemicals per sample of the composite biopolymers. Advantages of complementary employment in mass spectrometric methods and Fourier transform infrared spectroscopy is highlighted. The study utilizes ultra-high resolution electrospray ionization mass spectrometric and Fourier transform infrared spectroscopic data on biodegradable plastics bags for foodstuffs; high accuracy quantum chemical static methods, molecular dynamics; and chemometrics. There is achieved method performance |r| = 0.99981 determining poly(propylene glycol) in bag for foodstuff containing 20,416 species and using stochastic dynamics mass spectrometric formulas. The results highlight their great capability and applicability to the analytical science as well as relevance to both the fundamental research and to the industry.
Harnessing green chemistry for waste water remediation through Alpinia leaves silver nanoparticles
Vol 5, Issue 2, 2024
Download PDF
Abstract
Addressing environmental concerns with sustainable nanomaterial is a vital step towards innovative and eco-friendly techniques that address important global issues like pollution, water contamination, and resource depletion. This eco-friendly approach offers a sustainable alternative to conventional methods and this study aims to showcase the applications of biogenic silver nanoparticles (AgNPs) synthesized using green synthesis techniques with aqueous leaf extracts from five Alpinia varieties; Alpinia purpurata, Alpinia caerulea, Alpinia zerumbet ‘variegata’, Alpinia calcurata and Alpinia zerumbet. The AgNPs were synthesized using water extracts (WE) and silver nitrate at the optimum conditions. Characterization of AgNPs using UV-Vis spectroscopy and scanning electron microscopy (SEM), confirmed their successful formation and morphology. Spherical A.zerumbet_AgNPs between 28–68 nm in size were observed. The photocatalytic activity was tested by degrading methyl Orange (MO) dye under solar irradiation and the use NaBH4 with AgNPs significantly increased the degradation of MO. P-nitrophenol catalysis using AgNP and NaBH4 resulted promising results. Cytotoxicity of AgNPs using Artemia salina was evaluated and 100% viability was seen. The antibacterial activity was conducted using Escherichia coli and Staphylococcus aureus, unlike the WEs, all AgNPs showed antibacterial activity. This study revealed that the AgNPs synthesized using Alpinia leaves has diverse functional properties, presenting a promising avenue for future research and practical applications in environmental pollution.
Keywords
References
- Lu F, Astruc D. Nanocatalysts and other nanomaterials for water remediation from organic pollutants. Coordination Chemistry Reviews. 2020; 408. doi: 10.1016/j.ccr.2020.213180
- Singh A, Pal D, Mohammad A, et al. Biological remediation technologies for dyes and heavy metals in wastewater treatment: New insight. Bioresource Technology. 2024; 343. doi: 10.1016/j.biortech.2021.126154
- Malik S, Muhammad K, Waheed Y. Nanotechnology: A Revolution in Modern Industry. Molecules. 2023; 28(2): 661. doi: 10.3390/molecules28020661
- Abid N, Khan AM, Shujait S, et al. Synthesis of nanomaterials using various top-down and bottom-up approaches, influencing factors, advantages, and disadvantages: A review. Advances in Colloid and Interface Science. 2022; 300: 102597. doi: 10.1016/j.cis.2021.102597
- Singh NA, Narang J, Garg D, et al. Nanoparticles synthesis via microorganisms and their prospective applications in agriculture. Plant Nano Biology. 2023; 5: 100047–100047. doi: 10.1016/j.plana.2023.100047
- Ying S, Guan Z, Ofoegbu PC, et al. Green synthesis of nanoparticles: Current developments and limitations. Environmental Technology & Innovation. 2022; 26: 102336. doi: 10.1016/j.eti.2022.102336
- Kuthi NA, Basar N, Chandren S. Nanonutrition- and nanoparticle-based ultraviolet rays protection of skin. Elsevier eBooks; 2022. pp. 227–280. doi: 10.1016/b978-0-323-88450-1.00008-9
- Zhu X, Pathakoti K, Hwang HM. Green synthesis of titanium dioxide and zinc oxide nanoparticles and their usage for antimicrobial applications and environmental remediation. Green Synthesis, Characterization and Applications of Nanoparticles. 2019; 223–263. doi: 10.1016/b978-0-08-102579-6.00010-1
- Zhang Q, Zheng Y, Hu X, et al. Ethnopharmacological uses, phytochemistry, biological activities, and therapeutic applications of Alpinia oxyphylla Miquel: A review. Journal of Ethnopharmacology. 2018; 224: 149–168. doi: 10.1016/j.jep.2018.05.002
- Kumar KM, Asish G, Sabu M, Balachandran I. Significance of gingers (Zingiberaceae) in Indian System of Medicine-Ayurveda: An overview. Ancient Science of Life. 2013; 32(4): 253. doi: 10.4103/0257-7941.131989
- Priyono QAP, Yusniasari PA, Alifiansyah MRT, et al. Ethnomedical Potentials, Phytochemicals, and Medicinal Profile of Alpinia galanga L.: A Comprehensive Review. BIO Integration. 2024; 5(1). doi:doi: 10.15212/bioi-2024-0032
- Ray SS, Gusain R, Kumar N. Classification of water contaminants. Carbon Nanomaterial-Based Adsorbents for Water Purification. 2020; 11–36. doi: 10.1016/b978-0-12-821959-1.00002-7
- de Vasconcelos GMD, Della-Flora IK, Kelbert M, et al. Screening of Azo-Dye-Degrading Bacteria from Textile Industry Wastewater-Activated Sludge. Eng. 2024; 5(1): 116–132. doi: 10.3390/eng5010008
- Marimuthu S, Antonisamy AJ, Malayandi S, et al. Silver nanoparticles in dye effluent treatment: A review on synthesis, treatment methods, mechanisms, photocatalytic degradation, toxic effects and mitigation of toxicity. Journal of Photochemistry and Photobiology B: Biology. 2020; 205: 111823. doi: 10.1016/j.jphotobiol.2020.111823
- Celeste J, Mohan H, Sathya PM, et al. Detoxification of p-nitrophenol (PNP) using Enterococcus gallinarum JT-02 isolated from animal farm waste sludge. Environmental Research. 2023; 231: 116289–116289. doi: 10.1016/j.envres.2023.116289
- Kanimozhi S, Kanthimathi M. Green nanoparticles for industrially important reactions. Elsevier eBooks; 2023. pp.453–465. doi: 10.1016/b978-0-323-95921-6.00010-x
- Ahmed EM, Khaled HE, Elsayed A. Long-term exposure to p-Nitrophenol induces hepatotoxicity via accelerating apoptosis and glycogen accumulation in male Japanese quails. Environmental Science and Pollution Research. 2021; 28(32): 44420–44431. doi: 10.1007/s11356-021-13806-9
- Kuang S, Le Q, Hu J, et al. Effects of p-nitrophenol on enzyme activity, histology, and gene expression in Larimichthys crocea. Comparative Biochemistry and Physiology Part C: Toxicology & Pharmacology. 2020; 228, 108638. doi: 10.1016/j.cbpc.2019.108638
- Xu J, Wang B, Zhang W, et al. Biodegradation of p-nitrophenol by engineered strain. AMB Express. 2021; 11(1). doi: 10.1186/s13568-021-01284-8
- Chen J, Song M, Li Y, et al. The effect of phytosterol protects rats against 4-nitrophenol-induced liver damage. Environmental Toxicology and Pharmacology. 2015; 41: 266–271. doi: 10.1016/j.etap.2015.12.011
- Shimoga G, Palem RR, Lee S-H, Kim S-Y. Catalytic Degradability of p-Nitrophenol Using Ecofriendly Silver Nanoparticles. Metals. 2020; 10(12): 1661. doi: 10.3390/met10121661
- Bruna T, Maldonado-Bravo F, Jara P, Caro, N. Silver Nanoparticles and Their Antibacterial Applications. International Journal of Molecular Sciences. 2021; 22(13): 7202. doi: 10.3390/ijms22137202
- Loo YY, Rukayadi Y, Nor-Khaizura M-A-R, et al. In Vitro Antimicrobial Activity of Green Synthesized Silver Nanoparticles Against Selected Gram-negative Foodborne Pathogens. Frontiers in Microbiology. 2018; 9. doi: 10.3389/fmicb.2018.01555
- Badawy MEI, Lotfy TMR, Shawir SMS. Preparation and antibacterial activity of chitosan-silver nanoparticles for application in preservation of minced meat. Bulletin of the National Research Centre. 2019; 43(1). doi: 10.1186/s42269-019-0124-8
- Parihar A, Choudhary NK, Sharma P, et al. Evaluation of Electrochemical and Antimicrobial Efficacy of Green Synthesized Silver Functionalized Reduced Graphene Oxide Nanocomposites. Deleted Journal. 2024. doi: 10.1007/s44174-024-00185-1
- Liao C, Li Y, Tjong S. Bactericidal and Cytotoxic Properties of Silver Nanoparticles. International Journal of Molecular Sciences. 2019; 20(2): 449. doi: 10.3390/ijms20020449
- Rajabi S, Ramazani A, Hamidi M, Naji T. Artemia salina as a model organism in toxicity assessment of nanoparticles. DARU Journal of Pharmaceutical Sciences, [online] 23(1). doi: 10.1186/s40199-015-0105-x
- Libralatoa G, Prato E, Migliorec L, et al. A Review of Toxicity Testing Protocols and Endpoints with Artemia spp. Ecological Indicators. 2016; 69: 35–49. doi: 10.1016/j.ecolind.2016.04.017
- Chompoo J, Upadhyay A, Fukuta M, Tawata S. Effect of Alpinia zerumbet components on antioxidant and skin diseases-related enzymes. BMC Complementary and Alternative Medicine. 2012; 12(1). doi: 10.1186/1472-6882-12-106
- He Y, Wei F, Ma Z, et al. Green synthesis of silver nanoparticles using seed extract of Alpinia katsumadai, and their antioxidant, cytotoxicity, and antibacterial activities. RSC Advances. 2017; 7(63): 39842–39851. doi: 10.1039/C7RA05286C
- Ganapathy Selvam G, Sivakumar K. Phycosynthesis of silver nanoparticles and photocatalytic degradation of methyl orange dye using silver (Ag) nanoparticles synthesized from Hypnea musciformis (Wulfen) J.V. Lamouroux. Applied Nanoscience. 2014; 5(5): 617–622. doi: 10.1007/s13204-014-0356-8
- Saha P, Mahiuddin Md, Islam ABMN, Ochiai B. Biogenic Synthesis and Catalytic Efficacy of Silver Nanoparticles Based on Peel Extracts of Citrus macroptera Fruit. ACS Omega. 2021; 6(28): 18260–18268. doi: 10.1021/acsomega.1c02149.
- Carballo J, Hernández-Inda ZL, Pérez P, García-Grávalos MD. A comparison between two brine shrimp assays to detect in vitrocytotoxicity in marine natural products. BMC Biotechnology. 2002; 2(1): p.17. doi: 10.1186/1472-6750-2-17
- Filly A, Fabiano-Tixier AS, Louis C, et al. Water as a green solvent combined with different techniques for extraction of essential oil from lavender flowers. Comptes Rendus Chimie. 2016; 19(6): 707–717. doi: 10.1016/j.crci.2016.01.018
- Roy A, Bulut O, Some S, et al. Green synthesis of silver nanoparticles: biomolecule-nanoparticle organizations targeting antimicrobial activity. RSC Advances. 2019; 9(5): 2673–2702. doi: 10.1039/c8ra08982e
- Scholl JA, Koh AL, Dionne JA. Quantum plasmon resonances of individual metallic nanoparticles. Nature. 2012; 483(7390): 421–427. doi: 10.1038/nature10904
- Ismail R, Mubarak T, Al-Haddad R. Surface Plasmon Resonance of Silver Nanoparticles: Synthesis, Characterization, and Applications. J. Biochem. Tech. 2019; 10(2): 62–64.
- Pugazhendhi S, Kirubha E, Palanisamy PK, Gopalakrishnan R. Synthesis and characterization of silver nanoparticles from Alpinia calcarata by Green approach and its applications in bactericidal and nonlinear optics. Applied Surface Science. 2015; 357: 1801–1808. doi: 10.1016/j.apsusc.2015.09.237
- Tarannum N, Divya K, Gautam Y. Facile green synthesis and applications of silver nanoparticles: a state-of-the-art review. RSC Advances. 2019; 9(60): 34926–34948. doi: 10.1039/C9RA04164H
- Song JY, Kim BS. Rapid biological synthesis of silver nanoparticles using plant leaf extracts. Bioprocess and Biosystems Engineering. 2008; 32(1): 79–84. doi: 10.1007/s00449-008-0224-6
- Hodoroaba V-D, Rades S, Salge T, et al. Characterisation of nanoparticles by means of high-resolution SEM/EDS in transmission mode. IOP Conference Series: Materials Science and Engineering. 2016; 109: 012006. doi: 10.1088/1757-899x/109/1/012006
- Atmaca H, Pulat ÇÇ, Ilhan S. Synthesis of silver nanoparticles using Alpinia officinarum rhizome extract induces apoptosis through down-regulating Bcl-2 in human cancer cells. Biologia Futura. 2022; 73(3): 327–334. doi: 10.1007/s42977-022-00132-5
- Elbadawy HA, Elhusseiny AF, Hussein SM, Sadik WA. Sustainable and energy-efficient photocatalytic degradation of textile dye assisted by ecofriendly synthesized silver nanoparticles. Scientific Reports, Nature Portfolio. 2023. doi: 10.1038/s41598-023-29507-x
- Ajay S, Panicker JS, Manjumol KA, Subramanian PP. Photocatalytic activity of biogenic silver nanoparticles synthesized using Coleus Vettiveroids. Inorganic Chemistry Communications. 2022; 144: 109926. doi: 10.1016/j.inoche.2022.109926
- Sarkar S, Banerjee A, Halder U, et al. Degradation of Synthetic Azo Dyes of Textile Industry: a Sustainable Approach Using Microbial Enzymes. Water Conservation Science and Engineering. 2017; 2(4): 121–131. doi: 10.1007/s41101-017-0031-5
- Kyriakopoulos J, Kordouli E, Bourikas K, et al. Decolorization of Orange-G Aqueous Solutions over C60/MCM-41Photocatalysts. Applied Sciences. 2019; 9(9). doi: 10.3390/app9091958
- Jara YS, Mekiso TT, Washe AP. Highly efficient catalytic degradation of organic dyes using iron nanoparticles synthesized with Vernonia Amygdalina leaf extract. Scientific Reports. 2024; 14(1): 6997. doi: 10.1038/s41598-024-57554-5
- Bhankhar A, Giri M, Yadav K, Jaggi N. Study on degradation of methyl orange-an azo dye by silver nanoparticles using UV–Visible spectroscopy. Indian Journal of Physics. 2014; 88(11): 1191–1196. doi: 10.1007/s12648-014-0555-x
- Raj S, Singh H, Trivedi R, Soni V. Biogenic synthesis of AgNPs employing Terminalia arjuna leaf extract and its efficacy towards catalytic degradation of organic dyes. Scientific Reports. 2020; 10(1). doi: 10.1038/s41598-020-66851-8
- Gu S, Wunder S, Lu Y, et al. Kinetic Analysis of the Catalytic Reduction of 4-Nitrophenol by Metallic Nanoparticles. The Journal of Physical Chemistry C. 2014; 18618–18625. doi: 10.1021/jp5060606
- Al-Namil DS, Khoury EE, Patra D. Solid-State Green Synthesis of Ag NPs: Higher Temperature Harvests Larger Ag NPs but Smaller Size Has Better Catalytic Reduction Reaction. Scientific Reports. 2019; 9(1). doi: 10.1038/s41598-019-51693-w
- Dinda G, Halder D, Mitra A, et al. Phytosynthesis of silver nanoparticles using Zingiber officinale extract: evaluation of their catalytic and antibacterial activities. Journal of Dispersion Science and Technology. 2019; 41(14): 2128–2135. doi: 10.1080/01932691.2019.1653194
- Arulvasu C, Jennifer SM, Prabhu D, Chandhirasekar D. Toxicity Effect of Silver Nanoparticles in Brine ShrimpArtemia. The Scientific World Journal. 2014; 1–10. doi: 10.1155/2014/256919
- An HJ, Sarkheil M, Park HS, et al. Comparative toxicity of silver nanoparticles (AgNPs) and silver nanowires (AgNWs) on saltwater microcrustacean, Artemia salina. Comparative Biochemistry and Physiology Part C: Toxicology & Pharmacology. 2019; 218: 62–69. doi: 10.1016/j.cbpc.2019.01.002
- Osman AI, Zhang Y, Farghali M, et al. Synthesis of green nanoparticles for energy, biomedical, environmental, agricultural, and food applications: A review. Environmental Chemistry Letters. 2024. doi: 10.1007/s10311-023-01682-3
- Tongwanichniyom S, Phewrat N, Rangsarikorn N, et al. Green synthesis of silver nanoparticles using mature-pseudostem extracts of Alpinia nigra and their bioactivities. Green Processing and Synthesis. 2024; 13(1). doi: 10.1515/gps-2023-0226
- Lee SH, Jun B-H. Silver Nanoparticles: Synthesis and Application for Nanomedicine. International Journal of Molecular Sciences. 2019; 20(4): 865. doi: 10.3390/ijms20040865
- Urnukhsaikhan E, Bold B-E, Gunbileg A, et al. Antibacterial activity and characteristics of silver nanoparticles biosynthesized from Carduus crispus. Scientific Reports. 2021; 11(1): 21047. doi: 10.1038/s41598-021-00520-2
- Hossain MdM, Polash SA, Takikawa M, et al. Investigation of the Antibacterial Activity and in vivo Cytotoxicity of Biogenic Silver Nanoparticles as Potent Therapeutics. Frontiers in Bioengineering and Biotechnology. 2019; 7. doi: 10.3389/fbioe.2019.00239
- Bakhtiari-Sardari A, Mashreghi M, Eshghi H, et al. Comparative evaluation of silver nanoparticles biosynthesis by two cold-tolerant Streptomyces strains and their biological activities. Biotechnology Letters. 2020; 42(10): 1985–1999. doi: 10.1007/s10529-020-02921-1
- Imchen P, Ziekhrü M, Zhimomi BK, Phucho T. Biosynthesis of silver nanoparticles using the extract of Alpinia galanga rhizome and Rhus semialata fruit and their antibacterial activity. Inorganic Chemistry Communications. 2022; 109599. doi: 10.1016/j.inoche.2022.109599
Supporting Agencies
Copyright (c) 2024 Mathivathani Kandiah, Rathnayake P. Rathnayake, Beneli Gunaratne, Ominda Perera
License URL: https://creativecommons.org/licenses/by/4.0/
This site is licensed under a Creative Commons Attribution 4.0 International License (CC BY 4.0).
.jpg)
Beijing University of Technology, China