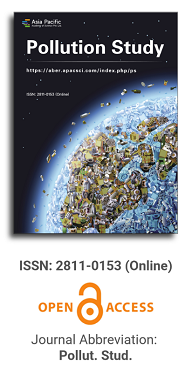
Asia Pacific Academy of Science Pte. Ltd. (APACSCI) specializes in international journal publishing. APACSCI adopts the open access publishing model and provides an important communication bridge for academic groups whose interest fields include engineering, technology, medicine, computer, mathematics, agriculture and forestry, and environment.
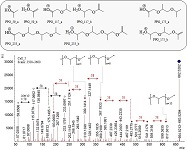
The (partial) replacement of synthetic polymers with bioplastics is due to increased production of conventional packaging plastics causing for severe environmental pollution with plastics waste. The bioplastics, however, represent complex mixtures of known and unknown (bio)polymers, fillers, plasticizers, stabilizers, flame retardant, pigments, antioxidants, hydrophobic polymers such as poly(lactic acid), polyethylene, polyesters, glycol, or poly(butylene succinate), and little is known of their chemical safety for both the environment and the human health. Polymerization reactions of bioplastics can produce no intentionally added chemicals to the bulk material, which could be toxic, as well. When polymers are used to food packing, then the latter chemicals could also migrate from the polymer to food. This fact compromises the safety for consumers, as well. The scarce data on chemical safety of bioplastics makes a gap in knowledge of their toxicity to humans and environment. Thus, development of exact analytical protocols for determining chemicals of bioplastics in environmental and food samples as well as packing polymers can only provide warrant for reliable conclusive evidence of their safety for both the human health and the environment. The task is compulsory according to legislation Directives valid to environmental protection, food control, and assessment of the risk to human health. The quantitative and structural determination of analytes is primary research task of analysis of polymers. The methods of mass spectrometry are fruitfully used for these purposes. Methodological development of exact analytical mass spectrometric tools for reliable structural analysis of bioplastics only guarantees their safety, efficacy, and quality to both humans and environment. This study, first, highlights innovative stochastic dynamics equations processing exactly mass spectrometric measurands and, thus, producing exact analyte quantification and 3D molecular and electronic structural analyses. There are determined synthetic polymers such as poly(ethylenglycol), poly(propylene glycol), and polyisoprene as well as biopolymers in bags for foodstuffs made from renewable cellulose and starch, and containing, in total within the 20,416–17,495 chemicals per sample of the composite biopolymers. Advantages of complementary employment in mass spectrometric methods and Fourier transform infrared spectroscopy is highlighted. The study utilizes ultra-high resolution electrospray ionization mass spectrometric and Fourier transform infrared spectroscopic data on biodegradable plastics bags for foodstuffs; high accuracy quantum chemical static methods, molecular dynamics; and chemometrics. There is achieved method performance |r| = 0.99981 determining poly(propylene glycol) in bag for foodstuff containing 20,416 species and using stochastic dynamics mass spectrometric formulas. The results highlight their great capability and applicability to the analytical science as well as relevance to both the fundamental research and to the industry.
Biomagnetic monitoring of the spatial distribution of atmospheric particulate matter in an industrialized city in Japan: Case study at Muroran
Vol 5, Issue 2, 2024
Download PDF
Abstract
Japan has 111 active volcanoes, supplying a great amount of magnetically-enhanced fly ashes. Such fly ashes likely mask anthropogenic magnetic signals; therefore, only a few magnetic biomonitoring studies have been reported in active volcanic regions, including Japan. The environmental magnetic results are reported for the materials deposited on Sasa Kurilensis, also known as dwarf bamboo, in the vicinity of the industrialized Muroran city center in Japan. The dust on the ten leaves at 105 sites was wiped off with a commercial wipe sheet, and their rock magnetic properties were analyzed. Room- and low-temperature magnetic analyses indicate that the major magnetic mineral in the dust is partially oxidized magnetite, ranging from single to pseudo single domain size, and the magnetic mineralogy on the leaves’ surface remains consistent throughout the study area. Much higher saturation isothermal remanent magnetization intensities are observed in the city’s eastern parts. The dominant wind directions in Muroran city are northwest, indicating that the steel companies in the city center are the major source of the fine-grained magnetic minerals on the dwarf bamboo leaves. These results indicate that using the leaves of dwarf bamboo for magnetic biomonitoring can be a non-destructive and rapid method to study the spatial distribution of atmospheric particulate matter from local industrial activities, even in active volcanic areas.
Keywords
References
- Evans M, Heller F. Environmental magnetism: principles and applications of enviromagnetics. Academic press; 2003
- Hofman J, Maher BA, Muxworthy AR, et al. Biomagnetic monitoring of atmospheric pollution: a review of magnetic signatures from biological sensors. Environmental Science & Technology. 2017, 51(12): 6648-6664.
- Heller F, Strzyszcz Z, Magiera T. Magnetic record of industrial pollution in forest soils of Upper Silesia, Poland. Journal of Geophysical Research: Solid Earth. 1998, 103: 17767-17774.
- Hoffmann V, Knab M, Appel E. Magnetic susceptibility mapping of roadside pollution. Journal of Geochemical Exploration. 1999, 66: 313-326.
- Könczöl M, Weiss A, Stangenberg E, et al. Cell-cycle changes and oxidative stress response to magnetite in A549 human lung cells. Chemical research in toxicology. 2013, 26: 693-702.
- Pankhurst Q, Hautot D, Khan N, et al. Increased levels of magnetic iron compounds in Alzheimer’s disease. Journal of Alzheimer’s disease. 2008, 13: 49-52.
- Brem F, Hirt AM, Winklhofer M, et al. Magnetic iron compounds in the human brain: a comparison of tumour and hippocampal tissue. Journal of The Royal Society Interface. 2006, 3: 833-841.
- Arosio P, Levi S. Ferritin, iron homeostasis, and oxidative damage. Free Radical Biology and Medicine. 2002, 33: 457-463.
- Maher BA, Ahmed IA, Karloukovski V, et al. Magnetite pollution nanoparticles in the human brain. Proceedings of the National Academy of Sciences. 2016, 113: 10797-10801.
- Fabian K, Reimann C, McEnroe SA, et al. Magnetic properties of terrestrial moss (Hylocomium splendens) along a north–south profile crossing the city of Oslo, Norway. Science of the Total Environment. 2011, 409: 2252-2260.
- Castañeda-Miranda AG, Chaparro MA, Pacheco-Castro A, et al. Magnetic biomonitoring of atmospheric dust using tree leaves of Ficus benjamina in Querétaro (México). Environmental Monitoring and Assessment. 2020, 192: 382.
- Zhang C, Huang B, Piper JD, et al. Biomonitoring of atmospheric particulate matter using magnetic properties of Salix matsudana tree ring cores. Science of the Total Environment. 2008, 393: 177-190.
- Kawasaki K, Horikawa K, Sakai H. Environmental Magnetism of Roadside Soil Contamination in the Restricted Bijyodaira Area of Mt. Tateyama, Toyama, Japan. Asian Journal of Water, Environment and Pollution. 2015, 12: 1-11.
- Kawasaki K, Horikawa K, Sakai H. Magnetic biomonitoring of roadside pollution in the restricted Midagahara area of Mt. Tateyama, Toyama, Japan. Environmental Science and Pollution Research. 2017, 24(11): 10313-10325.
- Kawasaki K, Fukushi K, Sakai H. Magnetic measurements of roadside topsoil pollution in an active volcanic region: Mt. Hakusan, Japan. Water and Environment Journal. 2018, 32(4): 556-565.
- Nakano S, Kaku S, Nakae K, et al. Relationship Between Industrial Development and City Planning in Company Towns of the Japanese Steel Industry During World War II A case study of Muroran, Kamaishi, Hirohata and Yahata. Urban and Regional Planning Review. 2016, 3: 163-186.
- Sawada Y. The Subsurface Geological Structure of the Alluvial Plain of Muroran, Hokkaido. Science reports of the Tohoku University. 2nd series, Geology. 1973. 6: 477-488
- Kakimoto H, Kitamura M, Matsumoto Y, et al. Comparison of atmospheric polycyclic aromatic hydrocarbons and nitropolycyclic aromatic hydrocarbons in Kanazawa, Sapporo and Tokyo. Journal of Health Science. 2000, 46(1): 5-15.
- Hayakawa K, Tang N, Morisaki H, et al. Atmospheric polycyclic and nitropolycyclic aromatic hydrocarbons in an iron-manufacturing city. Asian Journal of Atmospheric Environment. 2016, 10(2): 90-98.
- Symons DTA, Cioppa MT. Crossover plots: a useful method for plotting SIRM data in paleomagnetism. Geophysical Research Letters. 2000, 27: 1779-1782.
- Jordanova D, Jordanova N, Lanos P, et al. Magnetism of outdoor and indoor settled dust and its utilization as a tool for revealing the effect of elevated particulate air pollution on cardiovascular mortality. Geochemistry, Geophysics, Geosystems, 2012. 13: Q08Z49.
- Özdemir Ö, Dunlop DJ, Moskowitz BM. The effect of oxidation on the Verwey transition in magnetite. Geophysical Research Letters. 1993, 20: 1671-1674.
- Kosterov A. Low-temperature magnetization and AC susceptibility of magnetite: effect of thermomagnetic history. Geophysical Journal International. 2003, 154(1): 58-71.
- Moskowitz BM, Bazylinski DA, Egli R, et al. Magnetic properties of marine magnetotactic bacteria in a seasonally stratified coastal pond (Salt Pond, MA, USA). Geophysical Journal International. 2008, 174: 75-92.
- Verwey E, Haayman P. Electronic conductivity and transition point of magnetite (Fe3O4). Physica. 1941, 8: 979-987.
- Peters C, Dekkers M. Selected room temperature magnetic parameters as a function of mineralogy, concentration and grain size. Physics and Chemistry of the Earth, Parts A/B/C. 2003, 28(16): 659-667.
- Matsuo A, Tomimatsu H, Sangetsu Y, et al. Genet dynamics of a regenerating dwarf bamboo population across heterogeneous light environments in a temperate forest understorey. Ecology and Evolution. 2018, 8: 1746-1757.
- Petrovský E, Kapička A, Jordanova N, et al. Low-field magnetic susceptibility: a proxy method of estimating increased pollution of different environmental systems. Environmental Geology. 2000, 39(3): 312-318.
- Machemer SD. Characterization of airborne and bulk particulate from iron and steel manufacturing facilities. Environmental Science & Technology. 2004, 38(2): 381-389.
- Bućko MS, Mattila OP, Chrobak A, et al. Distribution of magnetic particulates in a roadside snowpack based on magnetic, microstructural and mineralogical analyses. Geophysical Journal International. 2013, 195(1): 159-175.
- Matzka J, Maher BA. Magnetic biomonitoring of roadside tree leaves: identification of spatial and temporal variations in vehicle-derived particulates. Atmospheric Environment. 1999, 33(28): 4565-4569.
- Lu S, Wang H, Guo J. Magnetic enhancement of urban roadside soils as a proxy of degree of pollution by traffic-related activities. Environmental Earth Sciences. 2011, 64(2): 359-371.
- Geological Survey of Japan, AIST (Ed.). Catalog of Eruptive Events during the Last 10,000 Years in Japan, Version 2.3. Geological Survey Japan, AIST. 2017.
- Moskowitz BM, Jackson M, Kissel C. Low-temperature magnetic behavior of titanomagnetites. Earth and Planetary Science Letters. 1998, 157: 141-149.
- Data collection of Asian Dust by Japan Meteorological Agency (in Japanese). Available online: https://www.data.jma.go.jp/gmd/env/kosahp/kosa_data_index.html (accessed on 2 October 2024).
- Stein AF, Draxler RR, Rolph GD, et al. NOAA’s HYSPLIT atmospheric transport and dispersion modeling system. Bulletin of the American Meteorological Society. 2015. 96: 2059-2077.
- Rolph G, Stein A, Stunder B. Real-time environmental applications and display system: READY. Environmental Modelling & Software. 2017, 95: 210-228.
- Kim W, Doh SJ, Yu Y. Asian dust storm as conveyance media of anthropogenic pollutants. Atmospheric Environment. 2012, 49: 41-50.
- Tsuchiya N, Kato S, Kawasaki K, et al. Sources of aeolian magnetite at a remote site in Japan: Dominantly Asian desert dust or anthropogenic emissions? Atmospheric Environment. 2023, 314: 120093.
- Li S, Zhang B, Wu D, et al. Magnetic particles unintentionally emitted from anthropogenic sources: iron and steel plants. Environmental Science & Technology Letters. 2021, 8(4): 295-300.
- Data collection of Past Weather by Japan Meteorological Agency (in Japanese). Available online: https://www.data.jma.go.jp/stats/etrn/index.php (accessed on 2 October 2024).
Supporting Agencies
Copyright (c) 2024 Kazuo Kawasaki, Nagisa Sawada
License URL: https://creativecommons.org/licenses/by/4.0/
This site is licensed under a Creative Commons Attribution 4.0 International License (CC BY 4.0).
.jpg)
Beijing University of Technology, China