Chemical sensors: From fundamentals to the future—A review
Tony Richard, Shiny Climensa
Article ID: 2838
Vol 5, Issue 2, 2024
DOI:
https://doi.org/10.54517/aas.v5i2.2838
VIEWS - 106
(Abstract)
Download PDF
Abstract
Chemical sensors bridge the gap between the chemical and electrical/optical domains, offering a powerful tool for analyzing our environment. These ingenious devices, with detection limits reaching parts-per-billion (ppb) for some analytes, rely on interactions between a specific material and the target molecule. This interaction, which can involve changes in electrical current, light emission, or mass, is translated into a measurable signal. This review delves into the core working principles of various sensor types, highlighting their diverse applications. From environmental monitoring (tracking air and water pollutants at concentrations as low as 10 ppb) to medical diagnostics (detecting biomarkers for early disease identification), chemical sensors play a crucial role in shaping a safer and healthier future. Recent advancements, such as miniaturization and integration with nanomaterials, promise even greater sensitivity, portability, and affordability, paving the way for a new era of sensor-driven innovation. This review article explores these advancements and their potential impact on various fields, inspiring further development and exploration of this transformative technology.
Keywords
chemical sensors; transduction; ultra-sensitive detection; miniaturization; biomedical diagnostics
Full Text
1. Introduction
The human experience has always been intertwined with the ability to sense the environment. Our biological senses—smell, taste, sight—provide invaluable information about the world around us. We can detect the acrid tang of smoke signaling fire, the sourness of spoiled food, or the color change of a litmus paper indicating a shift in acidity. However, these biological sensors are inherently limited. They lack the sensitivity to detect subtle changes in chemical composition, are easily overwhelmed by complex environments, and cannot differentiate between similar molecules. This is where chemical sensors emerge as powerful tools, extending our sensory reach and offering a more objective and quantitative understanding of the chemical world [1].
Chemical sensors [2] bridge the gap between the chemical and electrical/optical domains. These ingenious devices rely on a fascinating interplay of chemistry, physics, and engineering principles. At their core, chemical sensors utilize a specific material (receptor) that interacts with the target analyte (the chemical species of interest). This interaction can involve various mechanisms, such as adsorption (accumulation of the analyte on the sensor surface), a change in electrical conductivity, or the emission of light. The sensor then translates this interaction into a measurable signal—electrical current, change in voltage, or optical response—that can be quantified and interpreted.
The development of chemical sensors has been a remarkable journey. The early 20th century saw the invention of the rudimentary pH meter, a testament to the desire to move beyond subjective sensory experiences. Today, we have sophisticated biosensors capable of detecting specific molecules at trace levels, even in complex biological samples. These advancements are driven by the ever-increasing need for chemical sensing in our complex world [3].
Environmental pollution [4], a pressing global concern necessitates real-time monitoring of pollutants in air and water. Industrial processes demand precise control over chemical reactions to ensure product quality and safety. The field of medical diagnostics relies on rapid and accurate detection of biomarkers for early disease identification. Food safety hinges on vigilant monitoring for contamination and spoilage. Homeland security requires advanced detection of hazardous materials and explosives to safeguard public safety.
This review paper embarks on a captivating exploration of the world of chemical sensors, offering a thorough examination from foundational principles to cutting-edge applications. The paper will explore the core operating principles governing various sensor types, including electrochemical (relying on changes in electrical properties), optical (utilizing light-based interactions), mass-sensitive (measuring mass changes on the sensor surface), conductometric (measuring changes in electrical conductivity), piezoelectric (converting pressure changes into electrical signals), and thermal sensors (detecting changes in heat generation or absorption). Following this exploration of sensor types, the paper will examine their diverse applications in various fields: environmental monitoring (tracking air and water pollutants), industrial process control (ensuring efficient and safe operation), medical diagnostics (enabling rapid and accurate disease diagnosis), food safety and quality control (safeguarding the food supply and maintaining product quality), and homeland security and public safety (detecting hazardous materials and explosives).
However, this review aspires to be more than a simple compilation. A dedicated section will explore recent advancements in chemical sensor technology. This in-depth exploration of a specific cutting-edge area will be the key novelty of this paper compared to others. By providing a comprehensive overview, highlighting groundbreaking advancements, and focusing on a specific area of recent progress, this review paper aims to equip readers with a deeper understanding of the immense potential chemical sensors hold for shaping a safer, healthier, and more sustainable future.
2. Working principle
Chemical sensors [5] act like tiny translators, converting chemical interactions into measurable signals. At the heart of their operation lies a two-step process:
2.1. Recognition
The recognition element, often referred to as the receptor layer, is the heart of a chemical sensor. It’s where the magic happens—the initial interaction between the target analyte (the specific chemical you want to detect) and the sensor [6]. This interaction triggers a cascade of events, ultimately leading to a measurable signal. But how exactly does this recognition occur? Let’s delve deeper:
The Binding Ballet: Imagine the recognition layer as a dance floor and the analytes as dance partners. The sensor is designed to have a specific “dance style” in Mind—a particular way it interacts with its ideal partner (the target analyte). This “dance style” can take several forms:
Lock and Key: The recognition layer might have a specific molecular structure that perfectly fits the shape and functionality of the target analyte. This creates a strong, specific binding interaction, like a key fitting perfectly into a lock. Examples include antibodies designed to recognize specific proteins or enzymes with a high affinity for certain molecules.
Host-Guest Chemistry: The recognition layer may act like a host molecule, offering cavities or sites that can comfortably accommodate the target analyte (the guest). This creates a stable inclusion complex, much like a host welcoming a guest that fits perfectly within its designated space. This approach is often used in polymer-based sensors where specific functional groups within the polymer chain attract and bind the target analyte.
Beyond Binding: While binding is a common theme, recognition doesn’t always involve a physical attachment. In some cases, the analyte’s presence can alter the properties of the recognition layer itself, without forming a direct bond. Here are some examples:
Catalysts: The recognition layer may be a catalyst, a material that accelerates a chemical reaction. When the target analyte interacts with the catalyst, it can change the rate of the reaction, triggering a measurable signal. This is often used in gas sensors where the presence of a specific gas molecule alters the catalytic activity of a metal surface.
Swelling Materials: Some recognition layers are made of polymers that swell or contract upon exposure to specific analytes. This change in size can be measured and used as an indicator of the analyte’s presence. This approach is useful for detecting water vapor or organic solvents, which can cause swelling or shrinking of certain polymer films.
Designing for Selectivity: The key to an effective sensor is its selectivity—the ability to distinguish the target analyte from other potentially interfering chemicals in the environment. The design of the recognition layer plays a crucial role in achieving this. By carefully choosing the materials and tailoring their properties, scientists can create sensors that are highly specific to the target molecule.
For instance, an antibody-based sensor designed to detect a specific protein will have minimal interaction with other proteins present in a sample. Similarly, a sensor designed for a particular gas molecule will have a minimal response to other gases in the surrounding air.
The Importance of Recognition: The recognition element sets the stage for the entire sensing process [7]. By carefully designing this crucial component, scientists can create sensors that are sensitive, selective, and reliable—powerful tools for monitoring our environment, diagnosing diseases, and ensuring safety in various fields.
2.2. Transduction
The recognition layer may initiate the dance with the target analyte, but transduction is where the music truly starts [8]. It’s the process of converting the initial interaction between the analyte and the sensor into a measurable signal, like transforming a silent dance into a symphony. This signal allows us to “hear” what’s happening at the sensor level, revealing the presence and often the concentration of the target analyte. Here’s a breakdown of the different ways this conversion (transduction) can happen:
Electrochemical: The interaction with the analyte alters the sensor’s electrical properties, such as its conductivity or potential.
Optical: The interaction affects the way light interacts with the sensor material, leading to changes in absorption, reflection, or fluorescence.
Mass-Sensitive: The analyte binds to the sensor surface, causing a measurable change in mass.
Conductometric: The presence of the analyte alters the conductivity of a material within the sensor.
Piezoelectric: The interaction with the analyte induces a voltage in the sensor material due to the piezoelectric effect.
Thermal: The interaction with the analyte leads to a change in heat generation or absorption by the sensor.
By understanding the specific interaction between the analyte and the sensor material, scientists can design sensors with high selectivity, meaning they can distinguish the target analyte from other potentially interfering chemicals. This ability to selectively “smell” or “taste” specific chemicals.
3. Types of chemical sensors
The world of chemical sensors is vast and diverse, with each type offering unique strengths and applications [9]. Here, we’ll explore some of the most prominent categories based on their transduction mechanism:
3.1. Electrochemical sensors
Electrochemical sensors are the rockstars of the chemical sensor world, known for their sensitivity and versatility [10]. They translate chemical interactions at the electrode surface into electrical signals, allowing us to “hear” the whispers of target analytes in solution. Let’s delve deeper into how these sensors work and explore their different variations.
At the heart of every electrochemical sensor lies an electrode—a conductor that loves to exchange electrons with its surroundings. The recognition layer, often a thin film or coating on the electrode, plays a crucial role in this exchange. When a target analyte interacts with the recognition layer, it triggers a flow of electrons to or from the electrode. This change in electron flow forms the basis for the measurable signal.
Electrochemical sensors utilize various techniques to translate the electron flow into a quantifiable signal [11]. Here are three of the most common approaches:
Amperometric sensors: Imagine the target analyte acting like a conductor’s baton, orchestrating a flow of electrons at the electrode. Amperometric sensors measure the current (flow of electrons) generated by the chemical reaction between the analyte and the electrode. The higher the concentration of the analyte, the greater the current produced, allowing for sensitive detection. This technique is widely used in biosensors, where enzymes on the electrode surface catalyze reactions that generate measurable currents.
Potentiometric sensors: Here, the focus shifts to the voltage difference, like a conductor adjusting the dials to fine-tune the music. Potentiometric sensors measure the potential (voltage) difference between a working electrode (coated with the recognition layer) and a reference electrode (with a stable, constant potential). The presence of the analyte alters the potential at the working electrode, providing a signal for detection. Ion-selective electrodes (ISEs) are a prime example, where the recognition layer is highly selective for specific ions, leading to characteristic potential changes.
Conductometric sensors: Picture the analyte affecting the overall “conductivity” of the solution, like adding more instruments to an orchestra that alters the overall sound. This type of sensor measures the change in conductivity of the solution caused by the presence of ions in the analyte. As the concentration of the analyte increases, so does the number of ions in the solution, leading to a detectable change in conductivity. This approach is often used for simple and reliable detection of ionic contaminants in water or industrial process control applications.
Electrochemical sensors boast several advantages that make them popular choices for various applications:
1) High Sensitivity: They can detect minute amounts of analytes due to the precise measurement of electron flow.
2) Wide Range of Analytes: By tailoring the recognition layer, these sensors can detect a diverse range of chemicals, from ions and gases to biomolecules.
3) Fast Response Times: The electron transfer processes occur rapidly, allowing for real-time monitoring of chemical changes.
4) Compact and Portable Designs: Electrochemical sensors can be miniaturized for use in handheld devices or environmental monitoring equipment.
Unveiling the world through electrons:
From monitoring pollutants in water to detecting glucose levels in blood, electrochemical sensors play a vital role in various fields. Their ability to translate the silent language of chemical interactions into electrical signals makes them powerful tools for environmental monitoring, healthcare diagnostics, and industrial process control. As research continues, we can expect even more sophisticated electrochemical sensors to emerge, further revolutionizing our ability to understand and interact with the chemical world around us.
3.2. Optical sensors
Imagine a world where chemical interactions trigger a captivating light show—that’s the essence of optical sensors. These sensors use the power of light to detect the presence of analytes, transforming silent chemical conversations into a dazzling display. Let’s explore the fascinating world of optical sensors and delve deeper into some popular techniques [12].
Optical sensors rely on the interaction of light with the sensor material. When light encounters the sensor surface, it can be absorbed, reflected, or scattered in different ways [13]. The presence of an analyte can alter these interactions, leading to a detectable change in the light signal. This change is then measured and used to identify and quantify the target molecule.
Optical sensors employ a variety of techniques to translate light interactions into meaningful signals [14]. Here are some of the most common actors in this captivating play:
Surface Plasmon Resonance (SPR) Sensors: Imagine the analyte acting like a guest star, causing ripples in a sea of light. SPR sensors utilize a thin metal film that can couple with light waves, creating surface plasmons (collective electron oscillations). When an analyte binds to the sensor surface, it changes the refractive index (a measure of how light bends), altering the resonance conditions for surface plasmons. This shift in resonance is measured as a change in the reflected light intensity, revealing the presence of the analyte. SPR sensors are highly sensitive and often used for studying biomolecular interactions in real-time.
Fluorescence Sensors: Picture the analyte igniting a cascade of colorful fireworks. Fluorescence sensors use light-emitting molecules (fluorophores) embedded within the sensor material. When these fluorophores interact with the target analyte, they become excited and emit light at a different wavelength. The intensity or wavelength of the emitted fluorescence depends on the presence and concentration of the analyte, allowing for sensitive detection. This technique finds applications in immunoassays and biosensing, where specific antibodies labeled with fluorophores can detect target antigens.
Colorimetric Sensors: Imagine the analyte acting like a magic paintbrush, transforming the sensor’s color. Colorimetric sensors utilize indicator dyes that change color in response to specific chemical interactions with the analyte. This color change can be observed visually or measured using a light detector. These sensors are simple, inexpensive, and often used for point-of-care diagnostics or environmental monitoring applications. For instance, pH indicators change color based on the acidity or alkalinity of a solution.
The enchanting advantages of optical sensors:
Optical sensors offer several advantages that make them compelling choices for various applications:
1) High Sensitivity: They can detect minute changes in light interactions, leading to highly sensitive detection of analytes.
2) Real-Time Monitoring: Optical sensors allow for continuous monitoring of chemical changes, providing valuable insights into dynamic processes.
3) Label-Free Detection: Some techniques, like SPR, don’t require labeling the analyte with additional molecules, simplifying the analysis process.
4) Versatility: Optical sensors can be designed to detect a wide range of analytes by selecting appropriate light sources and detection methods.
From diagnosing diseases with biosensors to detecting pollutants in the environment, optical sensors play a crucial role in numerous fields. Their ability to translate chemical interactions into a captivating light show makes them powerful tools for medical diagnostics, environmental monitoring, and food safety analysis. As research progresses, we can expect even more sophisticated optical sensors to emerge, further illuminating our understanding of the chemical world.
3.3. Mass-sensitive sensors
Mass-sensitive sensors take a meticulous approach to chemical detection—they weigh the evidence! These fascinating devices measure the minute changes in mass on the sensor surface caused by the adsorption (attachment) of the target analyte [15]. This seemingly simple principle translates into highly sensitive and versatile sensors for a wide range of applications.
Imagine the sensor surface as a tiny, high-precision scale. When an analyte molecule interacts with the surface and binds, its weight adds to the overall mass [16]. Mass-sensitive sensors utilize a transducer material that converts this mass change into a measurable signal. Piezoelectric crystals are a popular choice—they vibrate at a specific frequency that is sensitive to changes in mass.
Here’s a breakdown of the process:
1) The Pristine Scale: The sensor starts with a clean surface and the piezoelectric crystal vibrates at its natural frequency.
2) The Analyte Arrives: When the target analyte enters the scene and binds to the surface, the mass of the sensor increases.
3) A Change in Tune: The added mass disrupts the vibration of the crystal, causing a shift in its frequency.
4) The Signal Emerges: This change in frequency is measured electronically and serves as the signal for analyte detection. The greater the frequency shift, the higher the concentration of the analyte.
Mass-sensitive sensors come in various forms, each with its strengths:
Quartz Crystal Microbalance (QCM) Sensors: These are the workhorses of the mass-sensitive world. They utilize a thin quartz crystal as the transducer material. As the analyte binds to the crystal surface, its resonant frequency changes, which is precisely measured and used to quantify the analyte. QCMs are highly sensitive and can detect a wide range of analytes, from biomolecules to vapors.
Surface Acoustic Wave (SAW) Sensors: These sensors operate on a similar principle to QCMs, but instead of crystals, they utilize surface acoustic waves on a piezoelectric material. The interaction with the analyte alters the velocity of these waves, which translates to a detectable signal. SAW sensors are known for their compact size and compatibility with microfluidic devices, making them suitable for miniaturized sensor applications.
The Enticing Advantages of Mass-Sensitive Sensors:
Mass-sensitive sensors offer several advantages that make them valuable for diverse applications:
High Sensitivity: They can detect minute changes in mass, allowing for highly sensitive and specific detection of analytes.
Label-Free Detection: These sensors often don’t require labeling the analyte with additional molecules, simplifying the analysis process.
Real-Time Monitoring: The changes in mass can be measured continuously, enabling real-time monitoring of chemical processes.
Broad Applicability: Mass-sensitive sensors can be tailored to detect a wide range of analytes in gas and liquid environments.
From monitoring air quality to studying biomolecular interactions, mass-sensitive sensors play a crucial role in various fields. Their ability to meticulously weigh the evidence of chemical interactions makes them valuable tools for environmental monitoring, food safety analysis, drug discovery, and fundamental research in chemistry and biology. As research advances, we can expect even more sophisticated mass-sensitive sensors to emerge, further refining our ability to measure and understand the chemical world at its most fundamental level.
3.4. Conductometric sensors
Conductometric sensors embody the beauty of simplicity in the realm of chemical detection. These workhorses rely on a fundamental property—the ability to measure changes in electrical conductivity—to reveal the presence of analytes. While they may not be the flashiest sensors around, their reliability and ease of use make them invaluable for industrial process control applications [17].
Imagine a solution like a bustling highway for charged particles called ions. The ease with which these ions can move through the solution determines its conductivity [18]. Conductometric sensors employ an electrode immersed in the solution. When an analyte enters the scene, it can:
Increase conductivity: If the analyte dissociates into ions in solution, it contributes to the overall flow of charged particles, leading to a rise in conductivity.
Decrease conductivity: In some cases, the analyte might hinder the movement of existing ions, resulting in a decrease in conductivity.
The sensor measures this change in conductivity, which can be directly linked to the presence and, in some cases, the concentration of the target analyte.
While the core principle remains the same, conductometric sensors can be categorized based on the type of conductive material used within the sensor:
Electrolytic Sensors: These sensors utilize a solution itself as the conductive material. The presence of the analyte alters the ionic composition of the solution, affecting its conductivity. This approach is often used for monitoring electrolytes in industrial processes or measuring the salinity of water.
Conductometric Gas Sensors: In these sensors, a metal oxide semiconductor acts as the conductive material. The interaction of gas molecules with the metal oxide surface changes its conductivity, allowing for the detection of specific gases. These sensors are commonly used for monitoring air quality or detecting leaks in industrial settings.
Conductometric sensors offer several advantages that make them particularly suitable for industrial applications:
Simplicity and Low Cost: The design is straightforward and requires minimal components, making them relatively inexpensive to manufacture and maintain.
Durability: These sensors are robust and can withstand harsh industrial environments.
Real-Time Monitoring: The changes in conductivity can be measured continuously, enabling real-time monitoring of chemical processes.
Wide Applicability: Conductometric sensors can be adapted to detect various analytes by selecting appropriate conductive materials and electrolytes.
Conductometric sensors find their niche in various industrial applications:
Monitoring Electrolyte Levels: In electroplating and battery production, maintaining the correct electrolyte concentration is crucial. Conductometric sensors ensure consistent quality control by continuously monitoring the conductivity of the electrolyte solution.
Detecting Leaks: These sensors can be used to detect leaks in pipelines carrying conductive solutions, preventing potential environmental or economic damage.
Monitoring Air Quality: Conductometric gas sensors help monitor air quality in industrial settings by detecting specific pollutants or changes in overall gas composition.
While conductometric sensors may not be the most glamorous, their reliable and straightforward approach to chemical detection makes them a vital cog in the machinery of various industries. As sensor technology continues to evolve, we can expect further advancements in materials and design to enhance the capabilities of these workhorse sensors.
3.5. Piezoelectric sensors
Piezoelectric sensors bring a unique melody to the world of chemical detection. They harness the fascinating piezoelectric effect, where mechanical stress on a material generates a voltage [19]. This allows them to translate the often-silent interaction between an analyte and the sensor surface into an electrical signal. While commonly used for pressure and vibration detection, piezoelectric sensors can also be fine-tuned for specific gas detection applications.
Imagine a piezoelectric material like a tiny microphone. When you apply pressure or stress, it vibrates, and interestingly, it also generates a voltage. This voltage is proportional to the applied stress. Piezoelectric sensors utilize this principle—the interaction with the target analyte creates stress on the sensor material, leading to a detectable voltage output [20].
Here’s a breakdown of the process:
1) The Serene Sensor: The piezoelectric crystal starts in a relaxed state, with no electrical output.
2) The Analyte Arrives: When a gas molecule interacts with the sensor surface (often coated with a selective material), it can cause adsorption (attachment) or induce chemical reactions.
3) A Song of Stress: This interaction creates stress or a change in the physical properties of the piezoelectric material.
4) The Voltage Emerges: The stress triggers a vibration within the crystal, and due to the piezoelectric effect, a voltage is generated. The magnitude of the voltage is directly related to the amount of stress caused by the analyte.
Not all gases create the same amount of stress on the piezoelectric material. The key to effective gas detection lies in tailoring the sensor for specific targets. Here’s how it’s achieved:
Selective Coatings: The piezoelectric crystal is often coated with a thin film that selectively interacts with the target gas molecule. This coating can be a polymer, a metal oxide, or another material that enhances the adsorption or reaction specific to the gas of interest.
Resonant Frequency: Piezoelectric crystals vibrate at a natural frequency. By carefully choosing the crystal size and design, scientists can tune the sensor to be most sensitive to the stress caused by the target gas molecule.
Piezoelectric sensors offer several advantages for gas detection applications
High Sensitivity: They can detect minute changes in stress, leading to sensitive detection of gas molecules.
Real-Time Monitoring: The voltage output can be measured continuously, enabling real-time monitoring of gas concentrations.
Durability: Piezoelectric materials are generally robust and can withstand harsh environments.
Compact Size: The sensors can be miniaturized, making them suitable for portable gas detection devices. Piezoelectric sensors play a crucial role in various gas detection applications
Air Quality Monitoring: These sensors can be used to monitor air quality in industrial settings or urban environments by detecting specific pollutants like volatile organic compounds (VOCs) or exhaust fumes.
Leak Detection: They can be used to detect leaks in pipelines carrying hazardous gases, ensuring safety and preventing environmental damage.
Breath Analysis: Piezoelectric sensors are being explored for breath analysis applications in medical diagnostics. By detecting specific biomarkers in exhaled breath, they might offer a non-invasive way to diagnose certain diseases.
As research progresses, we can expect further advancements in coatings and materials to enhance the selectivity and sensitivity of piezoelectric sensors, allowing them to play an even more prominent role in gas detection and analysis.
3.6. Thermal sensors
Thermal sensors take a different approach to chemical detection—they rely on the power of heat! These fascinating devices measure changes in temperature caused by the interaction between the analyte and the sensor material. This seemingly simple principle translates into a versatile tool for detecting combustible gases, monitoring exothermic reactions (reactions that release heat), and various other applications.
Imagine the sensor surface as a tiny thermometer, constantly monitoring its temperature. When an analyte interacts with the sensor, it can cause a change in temperature in two main ways:
Heat Generation: In some cases, the interaction between the analyte and the sensor material itself is exothermic, meaning it releases heat. This heat increase is measured by the sensor and serves as an indicator of the analyte’s presence.
Heat Absorption: Alternatively, the interaction might involve the analyte absorbing heat from the sensor. This heat loss is also detectable by the sensor and can be used to identify the analyte.
By measuring these subtle changes in temperature, thermal sensors can reveal the presence and sometimes even the concentration of the target molecule.
Thermal sensors come in various forms, each with its own strengths:
Catalytic Bead Sensors: These sensors utilize tiny beads coated with a catalyst—a material that accelerates chemical reactions. When a combustible gas molecule comes into contact with the catalyst, it undergoes a rapid reaction that releases heat. The sensor detects this heat increase, indicating the presence of the combustible gas. These sensors are commonly used in gas detectors for home safety or industrial settings.
Thermistor Sensors: Imagine a material that acts like a chameleon, changing its electrical resistance based on temperature. Thermistor sensors use such materials—their resistance increases as the temperature drops and vice versa. When the analyte interacts with the sensor, causing a change in temperature, the resistance of the thermistor material changes as well. This change in resistance is measured electronically and used to identify the analyte. Thermistor sensors are versatile and can be used for various applications, from monitoring exothermic reactions in laboratories to detecting air temperature changes in environmental monitoring systems.
Thermal sensors offer several advantages that make them valuable for specific applications:
Simplicity: The underlying principle is easy to understand, and the sensor design can be relatively straightforward.
Cost-Effectiveness: Thermal sensors can be manufactured at a lower cost compared to some other types of chemical sensors.
Sensitivity: They can be sensitive to minute changes in temperature, allowing for effective detection of certain analytes.
Wide Applicability: Different thermal sensor types cater to a variety of applications, from gas detection to monitoring reaction processes.
Thermal sensors find their niche in various applications:
Gas Detection: Catalytic bead sensors are widely used in household gas detectors, where they play a crucial role in safety by detecting combustible gases like methane (natural gas) or propane.
Process Monitoring: Thermistor sensors are valuable tools in laboratories and industrial settings for monitoring exothermic reactions. By tracking temperature changes, they ensure optimal reaction conditions and process efficiency.
Environmental Monitoring: Thermal sensors can be used to monitor air temperature changes or detect heat signatures associated with wildfires or industrial processes.
Thermal sensors represent just one facet of the fascinating world of chemical sensors. Each type—electrochemical, optical, mass-sensitive, conductometric, and piezoelectric—offers distinct advantages and limitations. Choosing the right sensor for a specific application depends on factors like the target analyte, desired sensitivity, and operational environment. As research continues, we can expect further advancements in thermal sensors and other types, leading to even more powerful and versatile tools for chemical detection and analysis.
4. Applications of chemical sensors
Chemical sensors play a vital role in various fields, ensuring safety, quality control, and a deeper understanding of our environment. Here, we’ll explore some key application areas:
4.1. Monitoring air and water quality
In our fight for a healthy planet, chemical sensors stand as vigilant guardians, constantly monitoring the air we breathe and the water we drink [21]. These remarkable devices act as our eyes and noses in the environmental realm, detecting a wide range of pollutants and helping us maintain a clean and sustainable future [22].
Air and water pollution pose significant threats to human health and ecological balance. Common air pollutants include:
Ozone (O3): A lung irritant that can trigger respiratory problems.
Nitrogen oxides (NOx): Contribute to smog formation and acid rain.
Volatile organic compounds (VOCs): Emitted from various sources, some VOCs are carcinogenic.
Water pollution encompasses a diverse range of contaminants, including:
Heavy metals: Lead, mercury, and arsenic can cause serious health problems even at low levels.
Industrial waste: Chemicals released from factories can disrupt aquatic ecosystems.
Agricultural runoff: Excess fertilizers and pesticides can contaminate water sources.
Chemical sensors play a vital role in environmental monitoring by:
Real-Time Detection: They provide continuous data on pollutant levels, enabling immediate response to pollution spikes.
Wide Range of Analytes: Different sensor types can detect a vast array of pollutants, from gases to heavy metals.
Portable and Compact Designs: Sensors can be deployed in various locations, including remote areas, for comprehensive environmental monitoring.
Here’s a closer look at how specific sensor types contribute to environmental
protection.
Electrochemical Sensors: Highly sensitive to nitrogen oxides and other air pollutants, these sensors are often used in stationary and mobile air quality monitoring stations.
Metal Oxide Semiconductor (MOS) Gas Sensors: These sensors can detect a broad spectrum of VOCs, making them valuable for monitoring industrial emissions or indoor air quality.
Ion-Selective Electrodes (ISEs): Specifically designed to detect ions of heavy metals like lead, mercury, and copper, ISEs are crucial for monitoring water quality in rivers, lakes, and industrial wastewater.
The data collected by chemical sensors is used for various purposes:
Enforcing Environmental Regulations: By tracking pollution levels, regulatory agencies can identify polluters and enforce environmental regulations.
Public Health Protection: Real-time air quality data can be used to warn vulnerable populations about smog events or high ozone levels.
Environmental Remediation Efforts: Sensor data helps target pollution sources and guide cleanup initiatives for contaminated water bodies.
The future of environmental monitoring holds promises for even more sophisticated chemical sensors. Here are some exciting possibilities:
Sensor Networks: Networks of interconnected sensors can provide comprehensive data on pollution levels across vast geographical areas.
Miniaturization and Lower Costs: Smaller and more affordable sensors will allow for wider deployment and citizen science initiatives.
Biosensor Development: Sensors utilizing biological elements can offer highly specific detection of emerging environmental contaminants.
By harnessing the power of chemical sensors, we can move towards a future with cleaner air, purer water, and a healthier planet for all. As technology advances, these remarkable devices will continue to be essential tools in our ongoing quest for environmental sustainability.
4.2. The silent orchestrators of industrial harmony
In the bustling world of industry, where efficiency and precision reign supreme, chemical sensors play a critical role as silent orchestrators [23]. These unsung heroes ensure smooth and efficient operation by constantly monitoring processes, safeguarding product quality, and preventing costly accidents. From the towering heights of chemical refineries to the intricate workings of pharmaceutical production lines, chemical sensors are the watchful eyes and sensitive noses that keep everything running smoothly.
Industrial processes are intricate dances of chemical reactions, each step requiring precise control for optimal results. Chemical sensors act as the conductors of this symphony, monitoring various parameters to ensure consistent product quality and efficient operation:
Reaction Progress Monitoring: Sensors can track the progress of chemical reactions in real time. By measuring factors like temperature, pH, or gas composition, they ensure reactions reach completion, maximizing yield and minimizing waste.
Quality Control: Sensors analyze the final product composition, identifying any deviations from specifications. This real-time feedback allows for adjustments to be made during production, preventing defective products from reaching the market.
Leak Detection: Chemical sensors can detect leaks of hazardous gases or liquids before they escalate into major incidents. This not only protects workers and the environment but also prevents costly product loss and production downtime.
Equipment Malfunction Prevention: Sensors can monitor parameters like vibration or temperature in machinery, potentially signaling equipment malfunctions before they occur. This proactive approach allows for preventive maintenance, minimizing downtime, and ensuring smooth operation.
The industrial world demands a diverse range of sensors to cater to various needs. Here are some of the key players:
Electrochemical Sensors: Widely used for monitoring pH, dissolved oxygen, and specific ions in solution, these sensors play a vital role in chemical processing and water treatment applications.
Mass Spectrometers: Offering unparalleled accuracy for identifying and quantifying complex mixtures, mass spectrometers are crucial for analyzing reaction products and ensuring final product quality in pharmaceutical and fine chemical industries.
Infrared (IR) Sensors: These sensors detect changes in the infrared radiation emitted by molecules, allowing for real-time monitoring of gas composition in industrial processes like combustion or fermentation.
The integration of chemical sensors into industrial processes offers numerous advantages
Enhanced Safety: Early leak detection and equipment malfunction prevention minimize the risk of accidents and environmental damage.
Improved Process Efficiency: Real-time monitoring allows for adjustments to optimize reaction conditions and minimize waste, leading to increased production yields.
Reduced Costs: By preventing accidents and ensuring consistent product quality, chemical sensors ultimately contribute to significant cost savings.
Improved Product Quality: Sensors ensure consistent product quality by providing real-time feedback on the production process.
The future of industrial process control promises even more sophisticated chemical sensors. Here are some exciting trends to watch:
Wireless Sensor Networks: Networks of interconnected sensors will provide a more comprehensive and real-time picture of entire production lines.
Miniaturization and Integration: Smaller and more integrated sensors will allow for wider deployment and closer monitoring of critical process parameters.
Advanced Materials and Detection Techniques: New materials and techniques will enable sensors with even higher sensitivity, selectivity, and faster response times.
By continuously innovating in the realm of chemical sensors, we can ensure a future where industrial processes become even more efficient, safe, and environmentally friendly. As these silent conductors continue to orchestrate industrial harmony, they pave the way for a more sustainable and productive future for the manufacturing sector.
4.3. Tiny detectives for big medical discoveries
The world of medicine is constantly evolving, and chemical sensors are emerging as powerful allies in the fight against disease. These miniature marvels are transforming medical diagnostics by offering faster, more convenient, and non-invasive ways to analyze samples, detect biomarkers, and monitor patients’ health [24].
Chemical sensors act as detectives in the human body, analyzing various aspects of our health:
Blood Gas Analysis: These sensors can measure critical blood gases like oxygen and carbon dioxide, providing valuable insights into respiratory function. This information is crucial for managing conditions like asthma or chronic obstructive pulmonary disease (COPD).
Biomarker Detection: Biomarkers are unique molecules in the body whose presence or levels can indicate disease. Chemical sensors can be designed to detect specific biomarkers, aiding in the early diagnosis of conditions like cancer, diabetes, or heart disease.
Glucose Monitoring: For diabetic patients, maintaining healthy blood sugar levels is vital. Chemical sensors embedded in continuous glucose monitoring (CGM) devices offer real-time monitoring, allowing patients to make informed decisions about their insulin intake and diet.
Chemical sensors offer several advantages in the realm of medical diagnostics:
Faster Results: Traditional diagnostic tests can be time-consuming. Chemical sensors provide quicker results, enabling faster treatment decisions.
Point-of-Care Testing: These sensors can be miniaturized and portable, allowing for on-site testing in doctor’s offices or even at home. This eliminates the need for lengthy hospital visits.
Non-invasive Techniques: Some sensors can analyze samples like sweat or breath, eliminating the need for painful or invasive procedures like blood draws.
Continuous Monitoring: Sensors like CGMs provide real-time data on a patient’s health, allowing for proactive management of chronic conditions.
The future of medical diagnostics with chemical sensors is brimming with potential:
Multiplexing Sensors: These sensors will be able to detect multiple biomarkers simultaneously, leading to a more comprehensive picture of a patient’s health.
Wearable Biosensors: Sensors integrated into wearable devices will allow for continuous monitoring of vital signs and health metrics.
Personalized Medicine: Sensors can be tailored to individual patients, enabling
personalized treatment plans based on their unique biomarkers and responses.
Chemical sensors are revolutionizing medical diagnostics by offering faster, more convenient, and less invasive ways to monitor health [25]. As sensor technology continues to evolve, we can expect even more sophisticated devices that will play a pivotal role in early disease detection, personalized medicine, and ultimately, improved patient care and well-being. By empowering healthcare professionals and patients with real-time health information, these tiny detectives hold the promise of a healthier future for all.
4.4. Guardians at the gate of food safety and quality
From farm to fork, chemical sensors play a critical role in safeguarding the food we consume. These vigilant guardians act as our eyes and noses in the food industry, ensuring safety by detecting spoilage, contamination, and freshness issues [26]. Additionally, they contribute to consistent product quality by monitoring key parameters like sugar content and acidity.
Chemical sensors stand guard against various threats to food safety:
Spoilage Detection: Sensors can detect volatile compounds released by deteriorating food, allowing for timely removal before spoilage becomes a major issue. This helps minimize food waste and ensures product quality.
Pathogen and Toxin Detection: Specific sensor types can be designed to identify harmful bacteria, viruses, or toxins that may contaminate food. This early detection prevents contaminated food from reaching consumers, safeguarding public health.
Freshness Monitoring: Sensors can measure parameters like gas composition or electrical conductivity, which correlate with food freshness. This information helps retailers and consumers make informed decisions about purchasing and consuming food products.
Chemical sensors are not just about safety; they also play a vital role in maintaining consistent food quality:
Sugar Content Measurement: Sensors can quickly and accurately measure sugar content in beverages, fruits, and other products. This ensures consistent sweetness perception and facilitates adherence to product specifications.
Acidity Monitoring: The pH level of food products significantly impacts taste and shelf life. Chemical sensors can monitor acidity throughout production, ensuring optimal product quality and taste.
Other Applications: Sensors can also measure fat content, moisture levels, and other parameters crucial for maintaining consistent quality across various food products.
The integration of chemical sensors into the food industry offers numerous advantages:
Enhanced Food Safety: Early detection of spoilage and contamination safeguards public health and minimizes the risk of foodborne illnesses.
Improved Quality Control: Consistent monitoring of key parameters ensures that food products meet quality standards and offer a predictable taste experience.
Reduced Food Waste: Timely detection of spoilage helps minimize food waste throughout the supply chain.
Streamlined Processes: Sensors can automate quality control procedures, leading to increased efficiency and reduced production costs.
The future of food safety and quality control holds promise for even more sophisticated chemical sensors. Here are some exciting possibilities to watch for:
Miniaturized and Portable Sensors: These sensors will allow for on-site testing of food products at various stages of the supply chain.
Multi-Analyte Sensors: Sensors capable of detecting multiple contaminants or quality parameters simultaneously will provide a more comprehensive picture of food safety and quality.
Wireless Sensor Networks: Networks of interconnected sensors will enable real-time monitoring of food storage and transportation conditions.
By harnessing the power of chemical sensors, we can move towards a future with a safer, tastier, and more reliable food supply. As sensor technology advances, these vigilant guardians will continue to play a vital role in safeguarding our food and ensuring a healthy and enjoyable dining experience for all.
4.5. Sentinels of security in a complex world
In the ever-evolving landscape of homeland security and public safety, chemical sensors stand as vigilant sentinels. These powerful tools act as the first line of defense, detecting hazardous chemicals, explosives, and biological agents that pose a threat to public safety [27]. From securing airports and critical infrastructure to safeguarding public spaces, chemical sensors play a vital role in preventing terrorist attacks and other security threats.
Chemical sensors act as the eyes and noses of security personnel, detecting a wide range of threats:
Chemical Warfare Agents: Sensors can be designed to detect nerve agents, blister agents, and other chemical weapons, allowing for rapid response and mitigation efforts in the event of an attack.
Explosives Detection: Sensor technology plays a crucial role in airport security, border crossings, and bomb disposal procedures. By detecting explosives at trace levels, these sensors help prevent catastrophic events.
Biological Agents: Specific sensors can identify bacteria, viruses, and other biological threats, enabling early intervention to prevent outbreaks and bioterrorism attacks [28].
Chemical sensors are deployed in various security applications:
Airport Security: These sensors are used to screen passengers and baggage for explosives and other harmful substances, ensuring safe air travel.
Critical Infrastructure Protection: Chemical sensors are vital for securing power plants, water treatment facilities, and other critical infrastructure from potential chemical or biological attacks.
Public Safety: Sensors can be deployed in public spaces like stadiums or mass transit systems to detect hazardous materials and ensure public safety during large gatherings or events.
The integration of chemical sensors into security protocols offers numerous advantages:
Enhanced Threat Detection: Early detection of hazardous materials allows for rapid response and minimizes potential damage.
Improved Public Safety: By securing airports, critical infrastructure, and public spaces, chemical sensors contribute significantly to a safer environment for all.
Streamlined Security Procedures: Sensors can automate screening processes, leading to faster security checks and improved efficiency.
Deterrence of Threats: The presence of sophisticated detection technology can deter potential attackers from attempting acts of terrorism or sabotage.
The future of homeland security and public safety holds exciting possibilities in the realm of chemical sensors:
Highly Selective Sensors: Sensors with even greater selectivity will be able to differentiate between harmless substances and specific threats with even higher accuracy.
Miniaturization and Portability: Smaller and more portable sensors will allow for wider deployment and on-site detection of threats in various scenarios.
Integrated Sensor Networks: Networks of interconnected sensors will provide real-time data on security threats across vast geographical areas.
Chemical sensors are not just tools for detection; they are vital instruments in shaping a safer and more secure future [29]. As sensor technology continues to evolve, we can expect even more innovative applications to emerge in various fields, contributing to a healthier, more sustainable, and ultimately, safer world for all.
4.6. Hydrogen production and domestic cooking applications
While chemical sensors haven’t yet become mainstream in monitoring hydrogen production and domestic cooking applications, their potential is significant. In hydrogen production [30,31], chemical sensors can enhance safety by detecting hydrogen leaks in pipelines and storage facilities using electrochemical or metal-oxide semiconductor sensors, which trigger alarms or initiate safety protocols upon detecting unsafe hydrogen levels [32,33]. They also ensure production quality by monitoring hydrogen purity during electrolysis or steam reforming, and prevent damage by detecting moisture intrusion in fuel cells. In domestic cooking with hydrogen-blended gaseous fuel [34,35], sensors are crucial for leak detection, requiring high sensitivity and rapid response times to ensure swift action. Commercially available combustible gas detectors, calibrated for blended fuels, can detect leaks, while flame monitoring sensors prevent gas build-up and potential explosions if the flame is extinguished accidentally [36]. Additionally, sensors may monitor combustion efficiency, optimizing cooking processes and reducing fuel consumption. Despite their promise, these applications face challenges like sensor selectivity, sensitivity, cost, durability, and the need for standardized protocols. Ongoing research aims to develop advanced sensors to address these challenges, ensuring safe and efficient hydrogen production and utilization, including for domestic cooking.
5. Recent advancements and future trends in chemical sensor technology
The field of chemical sensor technology is constantly evolving, driven by advancements in materials science, microfabrication, and data analysis [37]. Here’s a glimpse into some exciting trends:
Miniaturization and Integration: Sensors are becoming smaller, more portable, and often integrated with wireless communication capabilities, enabling real-time monitoring and remote data collection.
Enhanced Selectivity and Sensitivity: New materials and design strategies are leading to sensors with superior ability to distinguish target analytes from interfering chemicals, with even lower detection limits.
Multi-Analyte Detection: Sensors are being developed to detect multiple chemicals simultaneously, providing a more comprehensive picture of the chemical environment.
Smart Sensors: Sensors are being equipped with on-board processing capabilities, allowing them to perform data analysis and filtering at the sensor level, leading to smarter and more efficient operation.
These advancements pave the way for exciting future possibilities, such as:
Wearable Chemical Sensors: Sensors integrated into clothing or wearables for continuous health monitoring and environmental exposure assessment.
Biomimetic Sensors: Sensors inspired by nature, mimicking biological systems for highly selective and sensitive detection.
Sensor Networks: Networks of interconnected sensors creating a distributed sensing platform for real-time monitoring of large areas.
The future of chemical sensor technology is bright, promising to revolutionize how we interact with and understand the chemical world around us.
6. Conclusion
Chemical sensors have revolutionized our ability to interact with and understand the chemical world around us. They offer a powerful tool for extending our senses beyond their natural limitations, providing objective and quantitative data for diverse applications. This review paper explored the fundamental working principles of various sensor types, highlighting their unique strengths and functionalities. We delved into the extensive applications of chemical sensors in environmental monitoring, industrial process control, medical diagnostics, food safety, and homeland security, showcasing their impact on various aspects of our lives.
The future of chemical sensor technology is brimming with exciting possibilities. Recent advancements in areas like miniaturization, integration with nanomaterials, and enhanced selectivity hold immense potential for broadening sensor capabilities and applications. As research continues to push the boundaries, we can expect chemical sensors to become even more sensitive, versatile, and user-friendly. This will undoubtedly lead to further innovation and breakthroughs in various fields, contributing to a safer, healthier, and more sustainable future.
Conflict of interest: The authors declare no conflict of interest.
References
Korotcenkov G, Cho BK. Introduction to Chemical Sensor Technologies. Chemical Sensors: Comprehensive Sensor Technologies. Momentum Press; 2011.
Janata J. Chemical sensors. Analytical Chemistry. 1992; 64(12): 196–219. doi: 10.1021/ac00036a012
Paolesse R, Nardis S, Monti D, et al. Porphyrinoids for chemical sensor applications. Chemical Reviews. 2017; 117(4): 2517–2583.
Mimendia A, Gutiérrez JM, Leija L, et al. A review of the use of the potentiometric electronic tongue in the monitoring of environmental systems. Environmental Modelling & Software. 2010; 25(9): 1023-1030. doi: 10.1016/j.envsoft.2009.12.003
Janata J. Principles of Chemical Sensors. Springer US; 2009.
Cammann K, Lemke U, Rohen A, et al. Chemical sensors and biosensors—principles and applications. Angewandte Chemie International Edition in English. 1991; 30(5): 516–539.
Banica FG. Chemical sensors and biosensors: fundamentals and applications. John Wiley & Sons; 2012.
Wang J. Electrochemical transduction. Handbook of Chemical and Biological Sensors; 1996. doi: 10.1201/9781420050486.ch5
Vlasov YG, Ermolenko YE, Legin AV, et al. Chemical sensors and their systems. Journal of Analytical Chemistry. 2010; 65: 880–898.
Stetter JR, Penrose WR, Yao S. Sensors, Chemical Sensors, Electrochemical Sensors, and ECS. Journal of the Electrochemical Society. 2003; 150(2): S11. doi: 10.1149/1.1539051
Bakker E, Telting-Diaz M. Electrochemical Sensors. Analytical Chemistry. 2002; 74(12): 2781–2800. doi: 10.1021/ac0202278
Lobnik A, Turel M, Urek SK. Optical chemical sensors: design and applications. Advances in chemical sensors. 2012; 3(5).
Wolfbeis OS. Fiber-Optic Chemical Sensors and Biosensors. Analytical Chemistry. 2006; 78(12): 3859–3874. doi: 10.1021/ac060490z
Gauglitz G. Direct optical sensors: principles and selected applications. Analytical and Bioanalytical Chemistry. 2005; 381(1): 141–155. doi: 10.1007/s00216-004-2895-4
Gründler P. Mass-sensitive sensors. Chemical Sensors: An Introduction for Scientists and Engineers; 2007. 119–122.
Fischerauer G, Dickert FL. An analytic model of the dynamic response of mass-sensitive chemical sensors. Sensors and Actuators B: Chemical. 2007; 123(2): 993-1001. doi: 10.1016/j.snb.2006.11.002
Li D, Hu J, Wu R, et al. Conductometric chemical sensor based on individual CuO nanowires. Nanotechnology. 2010; 21(48): 485502. doi: 10.1088/0957-4484/21/48/485502
Steinberg MD, Žura I, Murkovic Steinberg I. Wireless smart tag with on-board conductometric chemical sensor. Sensors and Actuators B: Chemical. 2014; 196: 208–214. doi: 10.1016/j.snb.2014.02.012
Carey WP, Kowalski BR. Chemical piezoelectric sensor and sensor array characterization. Analytical Chemistry. 1986; 58(14): 3077–3084. doi: 10.1021/ac00127a037
Tressler JF, Alkoy S, Newnham RE. Piezoelectric sensors and sensor materials. J Electroceram. 1998; 2: 257–272.
Yaroshenko I, Kirsanov D, Marjanovic M, et al. Real-Time Water Quality Monitoring with Chemical Sensors. Sensors. 2020; 20(12): 3432. doi: 10.3390/s20123432
Kruse P. Review on water quality sensors. Journal of Physics D: Applied Physics. 2018; 51(20): 203002. doi: 10.1088/1361-6463/aabb93
Pérez-Gómez A. Attunement: Architectural meaning after the crisis of modern science. Mit Press; 2016.
Yao J, Yang M, Duan Y. Chemistry, Biology, and Medicine of Fluorescent Nanomaterials and Related Systems: New Insights into Biosensing, Bioimaging, Genomics, Diagnostics, and Therapy. Chemical Reviews. 2014; 114(12): 6130–6178. doi: 10.1021/cr200359p
Zhang X, Ju H, Wang J. Electrochemical sensors, biosensors and their biomedical applications. Academic Press; 2011.
Yang F, Yao J, Zheng F, et al. Guarding food safety with conventional and up-conversion near-infrared fluorescent sensors. Journal of Advanced Research. 2022; 41: 129–144. doi: 10.1016/j.jare.2022.01.011
Baraton MI. Sensors for environment, health and security: advanced materials and technologies. Springer Science & Business Media; 2008.
El-Safty SA, Shenashen MA. Nanoscale dynamic chemical, biological sensor material designs for control monitoring and early detection of advanced diseases. Materials Today Bio. 2020; 5: 100044. doi: 10.1016/j.mtbio.2020.100044
Philippe C, Anastasia M, Anastasios K, et al. Improving water quality and security with advanced sensors and indirect water sensing methods. Instrumentation and Measurement Technologies for Water Cycle Management. Springer; 2022. 251–277.
Aravindan M, Madhan Kumar V, Hariharan VS, et al. Fuelling the future: A review of non-renewable hydrogen production and storage techniques. Renewable and Sustainable Energy Reviews. 2023; 188: 113791. doi: 10.1016/j.rser.2023.113791
Aravindan M, Praveen Kumar G. Hydrogen towards sustainable transition: A review of production, economic, environmental impact and scaling factors. Results in Engineering. 2023; 20: 101456. doi: 10.1016/j.rineng.2023.101456
Aravindan M, Madhesh K, Praveen Kumar G, et al. Computational and Chemical Kinetics Analysis of Hydrogen-Blended LPG for Domestic Cook Stove Burners. Energy Conversion and Management: X. 2024; 22: 100568. doi: 10.1016/j.ecmx.2024.100568
Marimuthu A, Govindasamy PK. Driving an ecological transformation: unleashing multi-objective optimization for hydrogenated compressed natural gas in a decarbonization perspective. Environmental Science and Pollution Research. 2024; 31(21): 31632-31645. doi: 10.1007/s11356-024-33396-6
Aravindan M, Praveen Kumar G. Optimizing novel green hydrogen production from solar and municipal solid waste: A thermo-economic investigation with environmental comparison between integrated low temperature power cycles. Process Safety and Environmental Protection. 2024; 186: 421–447. doi: 10.1016/j.psep.2024.03.085
Aravindan M, Praveen Kumar G, Arulanandam MK, et al. Multi-objective optimization and analysis of chemical kinetics properties: Exploring the impact of different hydrogen blending ratios on LPG and methane-air mixtures. Energy Conversion and Management: X. 2024; 22: 100532. doi: 10.1016/j.ecmx.2024.100532
Aravindan M, Praveen Kumar G, Muthaiah R, et al. Unlocking clean gas with hydrogen: A combustion optimization study. Results in Engineering. 2024; 23: 102363. doi: 10.1016/j.rineng.2024.102363
Fang L, Jia M, Zhao H, et al. Molecularly imprinted polymer-based optical sensors for pesticides in foods: Recent advances and future trends. Trends in Food Science & Technology. 2021; 116: 387-404. doi: 10.1016/j.tifs.2021.07.039
Refbacks
- There are currently no refbacks.
Copyright (c) 2024 Tony Richard, Shiny Climensa
License URL:
https://creativecommons.org/licenses/by/4.0/
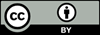
This site is licensed under a
Creative Commons Attribution 4.0 International License (CC BY 4.0).